Nuclear energy, also called atomic energy, is the powerful energy released by changes in the nucleus (core) of atoms. The heat and light of the sun result from nuclear energy. Scientists and engineers have found many uses for this energy. They include the production of electric power, the explosion of nuclear weapons, and the diagnosis and treatment of medical conditions.
Scientists knew nothing about nuclear energy until the early 1900’s, though they knew that all matter consists of atoms. Through further study, scientists learned that a nucleus makes up most of the mass (quantity of matter) of every atom. They also learned that this nucleus is held together by an extremely strong force. A huge amount of energy is concentrated in the nucleus because of this force. The next step was to make nuclei let go of much of that energy.
Scientists first released nuclear energy on a large scale at the University of Chicago in 1942, three years after World War II began. This achievement led to the development of the atomic bomb. The first atomic bomb was exploded in the desert near Alamogordo, New Mexico, on July 16, 1945. In August, United States planes dropped bombs on Hiroshima and Nagasaki, Japan. The bombs largely destroyed both cities and helped end World War II.
Since 1945, scientists have developed peaceful uses of nuclear energy. The energy released by nuclei creates large amounts of heat. This heat can be used to make steam. The steam, in turn, can be used to drive machines that generate electric power. Engineers have built devices called nuclear reactors to produce and control nuclear energy.
A nuclear reactor operates somewhat like a furnace. However, instead of using such fossil fuels as coal or oil, almost all reactors use uranium. And instead of burning in the reactor, the uranium fissions—that is, its nuclei split into two or more fragments. As a nucleus splits, it releases energy that is converted largely into heat. The fission of 1 pound of uranium releases more energy than the burning of 3 million pounds (1,500 tons) of coal. Stated in metric terms, the fission of 1 kilogram of uranium releases more energy than the burning of 3 million kilograms (3,000 metric tons) of coal.
One important peaceful use of nuclear energy is the production of electric power. Nuclear energy also powers some submarines, surface ships, and spacecraft. Nuclear reactions produce particles and rays called nuclear radiation that have uses in medicine, industry, and science. However, large doses of nuclear radiation can be extremely dangerous. Exposure to too much radiation can result in a condition called radiation sickness (see Radiation sickness).
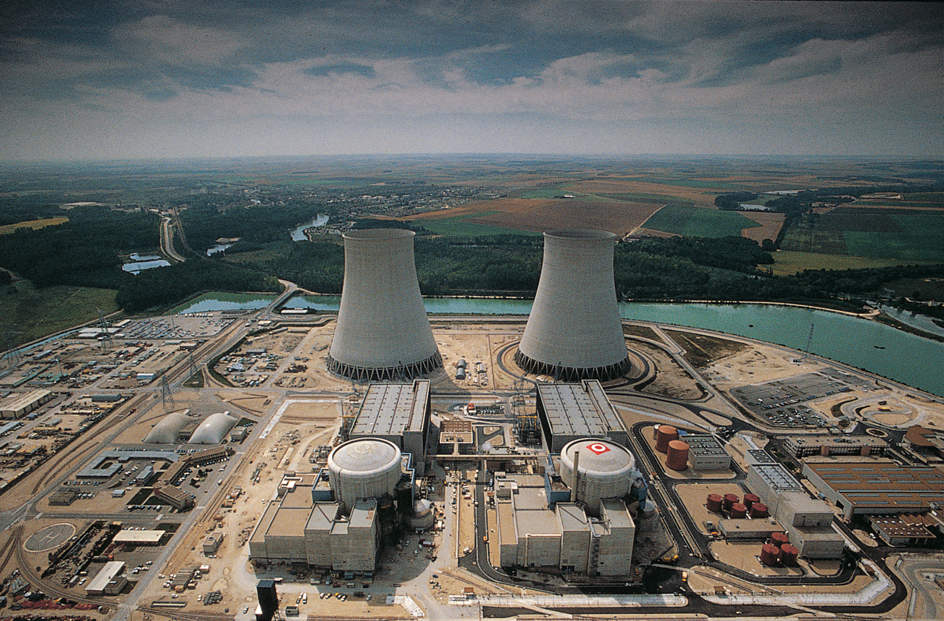
The role of nuclear energy in power production
Almost all the world’s electric energy is produced by hydroelectric or thermal power plants. Hydroelectric plants use the force of rushing water from a dam or waterfall to generate electric power. Thermal plants use the force of steam from boiling water. Most thermal plants burn fossil fuels—coal, oil, and natural gas—to produce heat to boil water. The remaining thermal plants fission uranium.
Few countries have enough water power to generate large amounts of hydroelectric power. Many regions have already fully exploited their hydroelectric capacity. Thus, most countries depend mainly on fossil fuels. But fossil fuels are a nonrenewable resource, and burning them produces gases that can damage the environment. Therefore, many experts predict that nuclear power will become increasingly important.
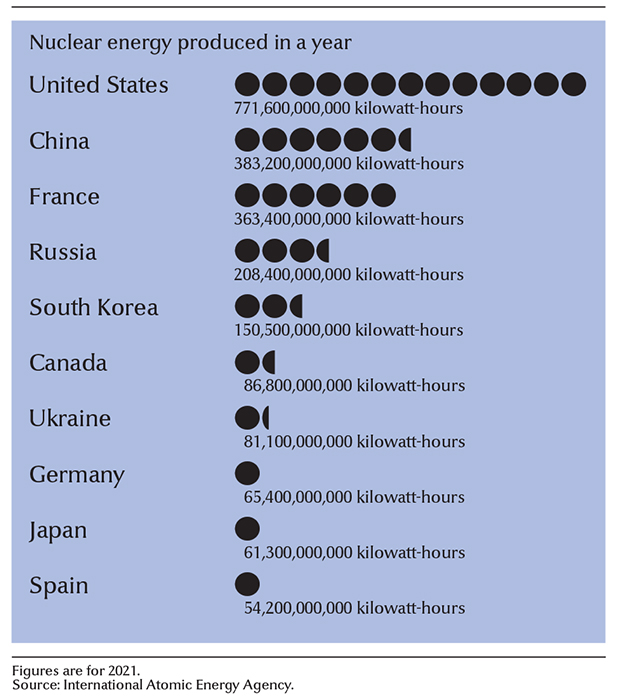
Worldwide use of nuclear energy.
Nuclear power reactors produce electric power in about 30 countries. There are about 440 reactors worldwide. These reactors produce about 10 percent of the world’s electric power. The United States is the world’s largest producer of nuclear energy. In addition to these reactors, there are more than 3,000 nuclear facilities worldwide that are used for applications in research, medicine, industry, and agriculture.
Advantages and disadvantages of nuclear energy.
Nuclear power plants have two main advantages over fossil-fuel plants. (1) Once built, a nuclear plant can be less expensive to operate than a fossil-fuel plant, mainly because a nuclear plant uses a much smaller volume of fuel. (2) Uranium, unlike fossil fuels, releases no chemical or solid pollutants into the air during use.
However, nuclear power plants have three major disadvantages. These disadvantages have slowed the development of nuclear energy in some countries. (1) Nuclear plants cost more to build than fossil-fuel plants. (2) Nuclear plants must meet stricter government regulations than do fossil-fuel plants. The regulations are meant to prevent hazardous amounts of radioactive material from being released. For example, a nuclear plant must satisfy the government that it can quickly and automatically deal with any kind of emergency. (3) Used nuclear fuel produces radiation long after it has been removed from the reactor. Some of the solid fission fragments are unstable and release radiation as they decay into other elements. As a result, safe disposal of nuclear waste presents a challenge.
The full development of nuclear energy.
Many experts believe that the benefits of nuclear energy outweigh any problems involved in its production. According to these experts, oil may be so scarce by the mid-2000’s that it will be too expensive to drill. Canada, China, Germany, India, Russia, the United States, and some other countries have enough coal to meet their energy requirements for hundreds of years at present rates of use. However, coal releases large amounts of sulfur and other pollutants into the air when it is burned (see Environmental pollution). If nuclear energy were fully developed, it could completely replace oil and coal as a source of electric power.
But a number of problems must be solved before nuclear energy can be fully developed. For example, almost all today’s power reactors use a scarce type of uranium known as U-235. If U-235 continues to be used at its present rate, the world’s supply of it will become so small that it will be too expensive to mine and process by 2100. Therefore, for nuclear energy to replace other energy sources in the future, it must be based on fuel that is much more plentiful than U-235.
The composition of matter
The process by which a nucleus releases energy is called a nuclear reaction. To understand the various types of nuclear reactions, a person must know something about the nature of matter.
All the matter that makes up all solids, liquids, and gases is composed of chemical elements. The chemical elements, in turn, are composed of atoms. A chemical element is a substance that cannot be broken down chemically into simpler substances. There are over 100 known chemical elements. Ninety-three of them are found on or in Earth, including very tiny amounts of an element called plutonium. All the other elements are artificially created.
Scientists rank the elements according to mass, a measure of the quantity of matter in an object. An object’s mass is proportional to its weight. Hydrogen is the lightest natural element, and plutonium is the heaviest. Most of the artificially created elements are heavier than plutonium.
Atoms and nuclei.
An atom consists of a positively charged nucleus and one or more electrons, which are negatively charged. The nucleus makes up almost all of an atom’s mass. The electrons, which have almost no mass, revolve about the nucleus. Electrons determine how an atom chemically reacts with other atoms (see Chemistry (Fundamental ideas of chemistry)). However, electrons do not play an active part in nuclear reactions.
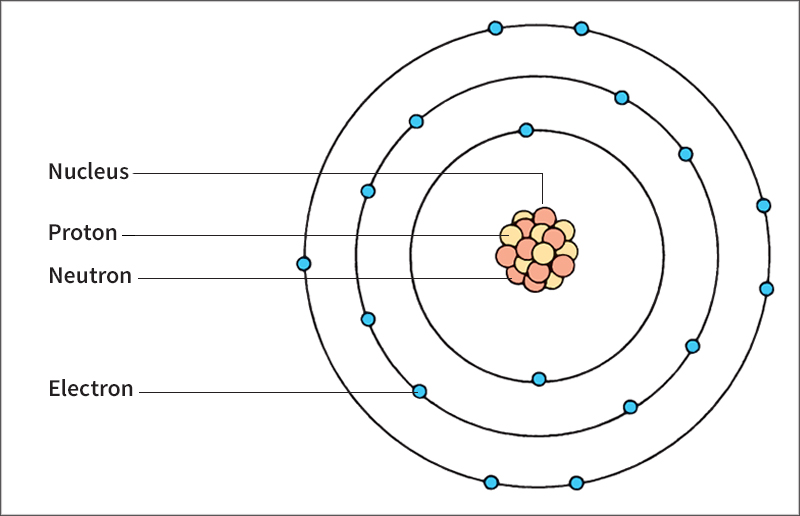
The nuclei of chemical elements consist of particles called protons and neutrons. An ordinary nucleus of hydrogen, the lightest element, has one proton and no neutrons. Heavier elements, such as uranium and plutonium, have large numbers of protons and neutrons.
Protons carry a positive charge. Neutrons have no net charge. Extremely strong forces, called nuclear forces, hold the protons and neutrons together in the nucleus. These nuclear forces determine the amount of energy required to release a nucleus’s neutrons and protons.
Isotopes.
Most chemical elements have more than one form. These different forms are called the isotopes of an element. An element’s various isotopes have the same number of protons, but different numbers of neutrons.
Scientists identify an isotope by its atomic mass number—that is, the total number of protons and neutrons in each of its nuclei. All the isotopes of a given element have the same number of protons in every nucleus. Every hydrogen nucleus, for example, has just 1 proton. Every uranium nucleus has 92 protons.
However, each isotope of an element has a different number of neutrons in its nuclei and so has a different atomic mass number. For example, the most plentiful isotope of uranium has 146 neutrons. Its atomic mass number is therefore 238 (the sum of 92 and 146). Scientists call this isotope uranium 238 or U-238. The uranium isotope that almost all nuclear reactors use as fuel has 143 neutrons, and so its atomic mass number is 235. This isotope is called uranium 235 or U-235.
No two elements have the same number of protons in their atoms. If an atom gains or loses one or more protons, it becomes an atom of a different element. However, if an atom gains or loses one or more neutrons, it becomes another isotope of the same element.
Nuclear reactions
A nuclear reaction changes the structure of a nucleus. The nucleus gains or loses one or more neutrons or protons. It thus changes into the nucleus of a different isotope or a different element. If the nucleus changes into the nucleus of a different element, the change is called a transmutation (see Transmutation of elements).
Three types of nuclear reactions release useful amounts of energy. These reactions are (1) radioactive decay, (2) nuclear fission, and (3) nuclear fusion. During each reaction, the matter involved loses mass. The mass is lost because it changes into energy.
Radioactive decay,
or radioactivity, is a process by which a nucleus changes into the nucleus of another isotope or element. The process releases energy chiefly in the form of particles and rays called nuclear radiation. Many elements have isotopes that are stable, meaning they cannot decay. But radioactive uranium, thorium, and several other elements are unstable and decay naturally. They contribute to the natural, or background, radiation that is always present on Earth. Nuclear reactors produce radioactive isotopes artificially. Nuclear radiation accounts for about 10 percent of the energy produced in a reactor.
Nuclear radiation consists largely of alpha particles, beta particles, and gamma rays. An alpha particle, which is made up of two protons and two neutrons, is identical to a helium nucleus. A beta particle is simply an electron. It results from the breakdown of a neutron in a radioactive nucleus. The breakdown also produces a proton, which remains in the nucleus. Gamma rays are electromagnetic waves similar to—but more powerful than—X rays (see Electromagnetic waves).
Scientists measure the rate of radioactive decay in units of time called half-lives. A half-life equals the time required for half the atoms of a particular radioactive element or isotope to decay. Half-lives range from a fraction of a second to billions of years. See Isotope (Radioactive isotopes).
Nuclear fission
is the splitting of heavy nuclei to release energy. All commercial nuclear reactors produce energy in this way. The two atomic bombs dropped on Japan in World War II used nuclear fission.
To produce fission, a reactor requires a bombarding particle, such as a neutron, and a target material, such as U-235. Nuclear fission occurs when the bombarding particle splits a nucleus in the target material into two or more parts called fission fragments. Each fragment consists of a nucleus with about half the neutrons and protons of the original nucleus. The energy is released in many forms. But most of the energy released by fission eventually takes the form of heat in the surrounding matter.
The bombarding particle
must first be captured by a nucleus for fission to occur. Reactors use neutrons as bombarding particles. They are the only atomic particles that are both easily captured and able to cause fission. Neutrons can also pass through most kinds of matter, including uranium.
The target material.
Commercial power reactors use uranium as their target material, or fuel. Uranium has one of the easiest nuclei to split because of its large number of protons. Protons naturally repel one another, and so a nucleus with many protons has a tendency to “fly apart.” It can thus be split with little difficulty.
Uranium also makes a good nuclear reactor fuel because it can sustain a continuous series of fission reactions. As a result, uranium can produce a steady supply of energy. To create a series of reactions, each fissioned nucleus gives off neutrons. Each of these neutrons can split another uranium nucleus, thus releasing still more neutrons. As this process is repeated over and over, it becomes a self-sustaining chain reaction. Chain reactions can produce an enormous amount of energy. Only nuclei that have many more neutrons than protons, such as uranium nuclei, can produce a nuclear chain reaction.
Nuclear power plants rely on the scarce uranium isotope U-235. It is the only natural material that nuclear reactors can use to produce a chain reaction. Nuclei of the much more abundant U-238 isotope usually absorb neutrons without fissioning. An absorbed neutron simply becomes part of the U-238 nucleus.
Neutrons released in fission travel too rapidly to be absorbed by U-235 nuclei in numbers large enough to sustain a chain reaction. Reactors can use U-235 as a fuel because they utilize other materials called moderators. The moderators slow the neutrons down, making nuclear fission much more likely to occur. Some reactors use water as a moderator, while others use graphite or heavy water. Heavy water contains an isotope of hydrogen called deuterium. A normal hydrogen nucleus has no neutrons, but deuterium has one neutron. Deuterium is also known as heavy hydrogen.
The slowed neutrons travel at a velocity of about 2.2 kilometers per second and are known as thermal neutrons. Reactors that use moderators are called thermal reactors. The vast majority of today’s reactors are thermal reactors.
Thermal neutrons are highly effective in causing fission in U-235. Therefore, the uranium in a thermal reactor can have a low percentage of U-235 content. Depending on their design, modern power reactors use a U-235 content ranging from 0.71 percent—the percentage in natural uranium—to almost 5 percent. Special purpose reactors may use fuel with a higher percentage of U-235.
Scientists have also developed fast reactors, in which high-velocity neutrons cause fissions without the use of a moderator. These reactors use plutonium or uranium 233 fuel. Fast breeder reactors produce more fuel material than they consume. A fast breeder reactor that converts U-238 to plutonium can greatly extend the use of uranium as an energy resource. In addition, a fast reactor can be designed to consume certain radioactive elements that have long lives and are present in used fuel. Such a reactor would reduce the amount of certain radioactive wastes that must be disposed of. The section Research on new types of reactors in this article discusses fast reactors in more detail.
Nuclear fusion
occurs when two lightweight nuclei fuse (combine) and form a nucleus of a heavier element. The products of the fusion reaction have less mass than the original nuclei had. The lost mass has therefore been changed into energy.
Fusion reactions that produce large amounts of energy require extremely intense heat. Such reactions are called thermonuclear reactions. The sun’s energy comes from thermonuclear reactions. Thermonuclear weapons—also called hydrogen bombs—are typically much more powerful than fission weapons. But scientists have not yet succeeded in harnessing the energy of fusion to produce electric power.
A thermonuclear reaction can only occur in a form of matter called plasma. Plasma is a gaslike substance made up of free electrons and free nuclei (nuclei that have no electrons revolving about them). Normally, nuclei repel one another because of the positive charges of their protons. However, if a plasma containing lightweight atomic nuclei is heated many millions of degrees, the nuclei begin moving so fast that they overcome the force of repulsion and fuse. See Plasma.
Problems of controlling fusion.
In their fusion experiments, scientists generally work with plasmas that are made from isotopes of hydrogen—protium, deuterium, and tritium. The most common hydrogen isotope, protium, has a nucleus that consists of a single proton. Deuterium is the second most common isotope. It has a nucleus made up of one proton and one neutron. The nucleus of the rarest isotope, tritium, consists of one proton and two neutrons. Tritium is not found in nature. Scientists produce tritium artificially by bombarding lithium nuclei with neutrons. Of the three isotopes of hydrogen, only tritium is radioactive.
A mixture of deuterium and tritium is an excellent thermonuclear fuel because ordinary seawater contains plentiful stocks of deuterium and lithium. One barrel of seawater contains enough of these substances to produce as much energy as the burning of about one-fifth of a barrel of oil.
To produce a controlled thermonuclear reaction, a plasma of one or more hydrogen isotopes must be heated many millions of degrees. Thermonuclear weapons—unlike nuclear reactors—quickly explode and so do not need to hold the hot plasma for an extended time. Scientists have yet to develop a container that can hold plasma this hot. The plasma expands quickly. In addition, the walls of the container must be kept at low temperatures to prevent them from melting. But if the plasma touches the walls, it becomes too cool to produce fusion. The plasma must therefore be kept away from the walls of the container long enough for its nuclei to fuse and produce usable amounts of energy.
Fusion devices.
Most experimental fusion reactors are designed to contain hot plasma in “magnetic bottles” twisted into various shapes. The walls of the bottles are made of copper or some other metal and are surrounded by electromagnets. Plasma, unlike gas, responds strongly to magnetic fields. The electromagnets can thus push the plasma away from the walls. All the fusion devices thus far developed, however, use much more energy than they create. The section Research on new types of reactors discusses experimental fusion reactors in greater detail.
How nuclear energy is produced
All large commercial nuclear power plants produce energy by fissioning U-235. But U-235 makes up only about 0.71 percent of the uranium found in nature. About 99.28 percent of all natural uranium is U-238. The two types occur together in uranium ores, such as carnotite and pitchblende. Separating the U-235 from the U-238 in these ores is difficult and costly. For this reason, the fuel used in reactors consists largely of U-238. But the fuel has enough U-235 to produce a chain reaction. Nuclear fuel requires special processing before and after it is used. The processing begins with the mining of uranium ore and ends with the disposal of fuel wastes.
This section deals chiefly with the methods used in the U.S. nuclear power industry. These methods resemble those used in other countries.
Power plant design.
Most nuclear power plants cover an area of 200 to 300 acres (80 to 120 hectares). The majority are built near a large river or lake because nuclear plants require enormous quantities of water for cooling purposes.
A nuclear plant consists of several main buildings, one of which houses the reactor and its related parts. Another main building houses the plant’s turbines and electric generators. Every plant also has facilities for storing unused and used fuel. Many plants are largely automated. Each of these plants has a main control room, which may be in a separate building or in one of the main buildings.
The reactor building, or containment building, has a thick concrete floor and walls of steel or of concrete lined with steel. The concrete and steel guard against the escape of radioactive material from an accidental leak in the nuclear reactor.
Power reactors
used in U.S. nuclear power plants consist of three main parts: (1) a reactor vessel, also called a pressure vessel; (2) a core; and (3) a set of control rods. In addition, reactor operations depend upon two substances—moderators and coolants.
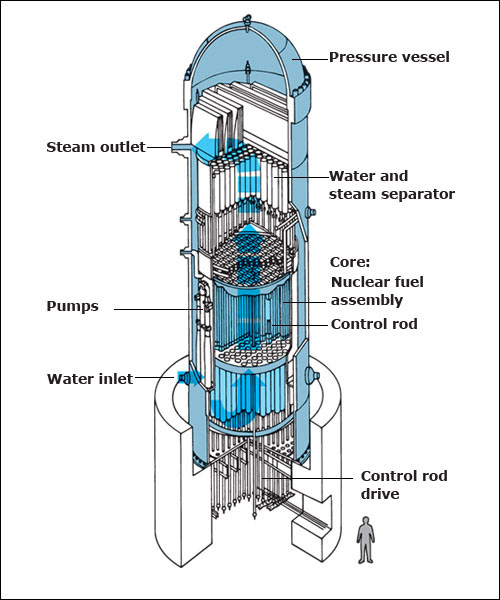
The reactor vessel is a tanklike structure that encloses the other main parts of the reactor. The vessel has steel walls that are typically up to at least 6 inches (15 centimeters) thick and capable of containing the high pressure exerted in a reactor.
The core contains the nuclear fuel in which the fission chain reaction occurs. The core sits in the lower half of the reactor vessel. A great many fuel assemblies stand upright in the core between an upper and lower support plate. Each fuel assembly contains a bundle of fuel rods. A fuel rod consists of pellets of fuel inside a metal tube. The pellet material is usually a dense powder made of uranium dioxide. The tubing material is typically zircaloy, a mixture of the metal zirconium and one or more other metals. Neutrons can pass from the fuel through the tube walls, but most other nuclear particles cannot.
The control rods are long metal rods that are used to regulate fission in the fuel. The control rods contain such neutron-absorbing materials as boron, cadmium, hafnium, or silver. A mechanism outside the reactor vessel is attached to the rods. This mechanism inserts the rods into the core and withdraws them when necessary. When inserted fully into the core, the control rods absorb many neutrons and so prevent a fission chain reaction from occurring. To begin operation of the reactor, the control rods are partially withdrawn until a chain reaction occurs at a constant rate. To increase power in the reactor, the rods are withdrawn slightly more. Thus, fewer neutrons are absorbed, and more are available to cause fission. To stop the chain reaction, the rods are inserted all the way into the core to absorb most of the neutrons.
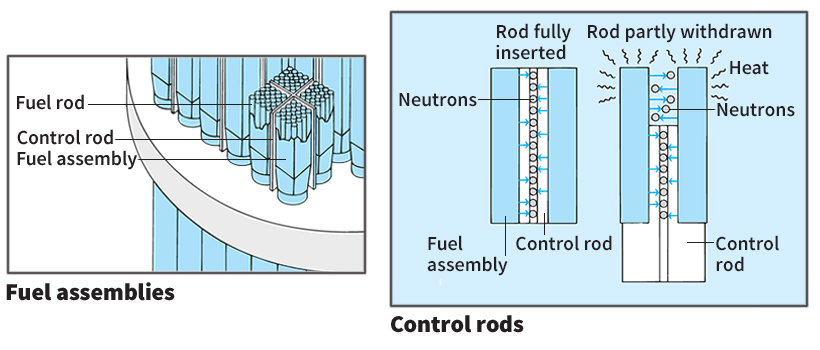
The moderator slows down neutrons as they pass through it, making fission more likely. The moderator fills the space between the fuel rods in the fuel assemblies.
The coolant is a liquid or gas that carries off the heat created by the fission chain reaction. The coolant circulates throughout the core. It carries the heat from the reactor to an energy conversion system. Thus, the coolant keeps the fuel rods from getting too hot. It also transfers energy to a place where electric power can be generated.
All commercial power reactors in the United States are light water reactors. In these devices, light (ordinary) water serves as the moderator and the coolant. Canadian reactors are heavy water reactors. They use heavy water as the moderator and the coolant. Heavy water contains deuterium in place of ordinary hydrogen. For more information on reactors, see the section “Research on new types of reactors” in this article.
Fuel preparation.
After uranium ore has been mined, it goes through a long milling and refining process. The process separates the uranium from other elements in the ore. Light water absorbs more neutrons than do other types of moderators. The uranium used in light water reactors must therefore be enriched—that is, the percentage of U-235 must be increased. Neutrons then have a better chance of striking a U-235 nucleus. In the United States, uranium that has been separated from the ore is sent to an enrichment plant.
Enrichment plants increase the proportion of U-235 in the uranium, depending on the intended use of the uranium. Most light water reactors use fuel with about 2 to 4 percent U-235. Much higher amounts of U-235 are needed in nuclear weapons and in the fuel for nuclear ships. The enriched uranium is shipped to fuel fabrication plants to make fuel rods.
A fuel fabrication plant changes enriched uranium into uranium dioxide. The plant then shapes the uranium dioxide powder into pellets about 1/3 inch (8 millimeters) in diameter and 1/2 inch (13 millimeters) long. The pellets are inserted into tubes made of zircaloy. Each tube measures about 1/2 inch (13 millimeters) in diameter and 10 to 14 feet (3 to 5 meters) long.
After a tube has been filled with uranium dioxide pellets, its ends are welded shut. This sealed tube is called cladding. These fuel rods are then fastened together into bundles of 30 to 300 each. Each bundle, or fuel assembly, weighs 300 to 1,500 pounds (140 to 680 kilograms). Commercial power reactors need 50 to 150 tons (45 to 136 metric tons) of uranium dioxide. The amount depends on the size of the reactor.
Chain reactions.
A reactor requires a certain minimum amount of fuel to keep up a chain reaction. This amount, called the critical mass, varies according to the design and size of the reactor. Reactors are designed to hold more than a critical mass of fuel to allow for fuel use during operation. The position of the control rods determines the effective mass of the fuel—that is, the amount of fuel taking part in the chain reaction. If the effective mass is decreased below the critical mass, the chain reaction will die out and reactor power will decrease. If the effective mass is increased above the critical mass, the chain reaction will become more rapid and reactor power will increase. In an emergency, if the chain reaction became too rapid, the reactor could overheat. However, the control rods are available to slow down the chain reaction if it becomes too rapid.
To prepare a reactor for operation, the fuel assemblies are loaded into the core with the control rods completely inserted. In a light water reactor, the water used as a moderator fills the spaces between the fuel assemblies. The control rods are then slowly withdrawn, and a chain reaction begins. The farther the rods are withdrawn, the greater the rate of the reaction because fewer neutrons are absorbed. More neutrons thus are available to cause fission. When the desired power is reached, the control rods are positioned so that the effective mass is equal to the critical mass. The water in the core carries off the heat created by the chain reaction. To stop the reaction, the rods are again inserted all the way into the core to absorb most neutrons.
Steam production.
The light water reactors used by almost all U.S. nuclear plants are of two main types. One type, the pressurized water reactor, produces steam outside the reactor vessel. The other type, the boiling water reactor, makes steam inside the vessel.
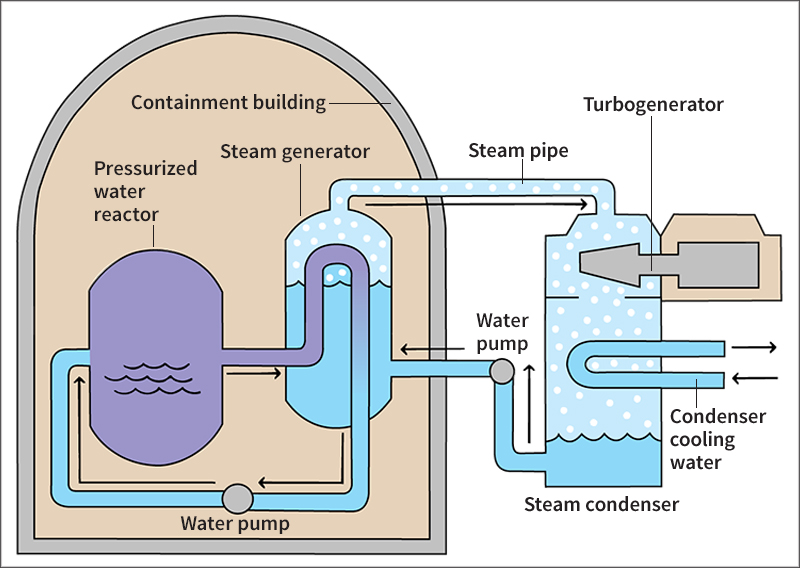
Most nuclear plants in the United States use pressurized water reactors. These reactors heat the moderator water in the core under extremely high pressure. The pressure allows the water to heat past its normal boiling point of 212 °F (100 °C) without actually boiling. The chain reaction heats the water to about 600 °F (316 °C). Pipes carry this extremely hot—though not boiling—water to steam generators outside the reactor. These generators transfer heat from the pressurized water to a separate supply of water that boils at a lower pressure and so produces steam.
In a boiling water reactor, the chain reaction boils the moderator-water in the core. Steam is therefore produced inside the reactor vessel. Pipes carry the steam from the reactor to the plant’s turbines.
In producing electric energy, a nuclear plant’s steam turbines and electric generators work like those in a fossil-fuel plant. The steam produced by a reactor spins the blades of the plant’s turbines, which drive the generators. Many plants have combination turbines and generators called turbogenerators.
After steam has passed through a plant’s turbines, it is piped to a condenser. The condenser transfers heat to a separate water stream and changes the steam back into water. A reactor can thus use the same water over and over. But a condenser requires a constant supply of cool, fresh water to cool the steam. Most plants pump this water from a nearby river or lake. The water, which becomes warm as it passes through the condenser, is then pumped back into the river or lake. This wastewater’s heat must be kept within limits so that the water in the river or lake does not endanger plants and animals that live there. The discharge of the warmer wastewater is sometimes called thermal pollution.
To minimize thermal pollution, new nuclear plants have cooling towers. Hot water from the steam condensers is moved through the towers in such a way that the heat passes into the atmosphere. Some water also evaporates. The cooled water is returned to the steam condenser for reuse.
Hazards and safeguards.
An ordinary power reactor cannot explode like an atomic bomb. Only a greatly supercritical mass of plutonium 239 or of highly enriched uranium 235 can explode in this way. A supercritical mass contains much more than the amount of plutonium or uranium required to sustain a chain reaction.
The chief hazards of nuclear power production result from the great quantities of radioactive material that a reactor produces, including fission products and transmuted materials in the reactor vessel. These materials give off radiation in the form of alpha and beta particles and gamma rays. The reactor vessel is surrounded by thick concrete blocks called a shield, which normally prevents almost all radiation from escaping.
Federal regulations limit the amount of radiation allowed from U.S. nuclear plants. Every plant has instruments that continually measure the radioactivity in and around the plant. They automatically set off an alarm if the radioactivity rises above a predetermined level. If necessary, the reactor is shut down.
A plant’s routine safety measures greatly reduce the possibility of a serious accident. Nevertheless, every plant has emergency safety systems that remove heat from radioactive products. Possible emergencies range from a break in a reactor water pipe to a leak of radiation from the reactor vessel. Any such emergency automatically activates a system that instantly shuts down the reactor, a process called scramming. The usual method of scramming is to insert the control rods rapidly in the core.
A leak or break in a reactor water pipe could have dangerous consequences if it results in a loss of coolant. Even after a reactor has been shut down, the radioactive materials remaining in the reactor core produce large amounts of heat. They can become so hot without sufficient coolant that the core melts. This condition, called a meltdown, could result in the release of dangerous amounts of radiation. In most cases, the large containment structure that houses a reactor would prevent radioactive material from escaping into the atmosphere. To prevent such an accident from occurring, all reactors are equipped with an emergency core cooling system, which automatically floods the core with water in case of a loss of coolant.
Wastes and waste disposal.
The fissioning of U-235 produces more neutrons than are needed to continue a chain reaction. Some of them combine with U-238 nuclei, which far outnumber U-235 nuclei in the reactor fuel. When U-238 captures a neutron, it is changed into U-239. The U-239 then decays into neptunium 239 (Np-239), which decays into plutonium 239 (Pu-239). This same process forms Pu-239 in a breeder reactor. Slow neutrons can fission Pu-239, as well as U-235. Some of the newly formed Pu-239 is thus fissioned during the fissioning of U-235. The rest of the Pu-239 remains in the fuel assemblies.
The fissioning of U-235 also produces many other radioactive isotopes, such as strontium 90, cesium 137, and barium 140. Fuel wastes can remain dangerously radioactive for hundreds of years because of the strontium and cesium isotopes. After that time, enough of the strontium and cesium will have decayed into stable isotopes so that they no longer present a severe problem. However, the plutonium and other artificially created elements from U-238 transmutation remain radioactive for thousands of years. Even in small amounts, plutonium can cause cancer or genetic damage in human beings. Larger amounts can cause radiation sickness and death (see Radiation sickness). Safe disposal of these wastes is one of the most difficult problems involved in nuclear power.
Most nuclear plants need to replace about a third of their fuel assemblies every year. The radioactive wastes generate heat, and so used fuel assemblies must be cooled after removal from a reactor. Nuclear plants cool the assemblies by storing them underwater in specially designed storage pools for several years. Later, the wastes are transferred to air-cooled concrete casks.
A storage site for nuclear waste must lie in a highly stable area that is free of earthquakes, faulting, and other geologic activity. The site must be dry so that the waste containers cannot be corroded and water supplies cannot be contaminated. The site also must be constructed so that future generations do not dig into it and release radioactivity. In 2002, the U.S. government selected Yucca Mountain, Nevada, as the country’s single nuclear waste storage site. In 2009, however, the government said that it no longer considered Yucca Mountain an option for storing nuclear waste after facing political opposition. In the meantime, commercial nuclear power plants in the United States continue to store used fuel assemblies and other wastes in pools of water and in concrete casks.
Other countries, including Japan, France, and Russia, are pursuing a reprocessing plan. Under this plan, nuclear plants would ship their used fuel assemblies to the reprocessing plants for removal of Pu-239 and unused U-235. These radioactive isotopes would then be recycled into fuel for nuclear reactors. However, this method would leave radioactive isotopes in the chemical solutions used for reprocessing. These solutions would have to be changed into a solid form that could be safely stored.
The nuclear energy industry
In every country that has a nuclear energy industry, the government plays a role in the industry. But the government’s role varies greatly among countries. This section deals mainly with the U.S. and Canadian nuclear energy industries.
Organization of the industry.
Private utility companies own most of the nuclear power plants in the United States. The rest are publicly owned. Private companies also manufacture reactors, mine uranium, and handle most other aspects of U.S. nuclear power production.
Canada’s nuclear power plants are all publicly owned. Atomic Energy of Canada Limited (AECL), a government corporation, has overall responsibility for the country’s nuclear research and development. AECL also designs the CANDU (CANada Deuterium oxide-Uranium) heavy water reactors used by all Canadian nuclear plants. Private companies make the various reactor parts and process the country’s uranium. Canada has no enrichment plants because CANDU reactors operate with natural uranium as fuel.
The industry and the economy.
The main economic advantage of nuclear power plants is that their fuel is less expensive than fossil fuels. But nuclear plants cost somewhat more to build than do fossil-fuel plants.
Under normal economic conditions, a nuclear plant’s savings in fuel eventually make up for its higher construction expenses. At first, these expenses add to the cost of producing electric power. But after some years, a plant will have paid off its construction costs. It can then produce electric power more cheaply than a fossil-fuel plant can.
The industry and the environment.
Unlike fossil-fuel plants, nuclear plants do not release solid or chemical pollutants into the atmosphere. For this reason, nuclear power is expected to play a major role in enabling several countries to reduce their emissions of various heat-trapping gases. However, a nuclear plant releases small amounts of radioactive gas into the air. In addition, the cooling water used in pressurized water plants picks up a small amount of radioactive tritium in the steam condenser. The tritium remains in this water when it is returned to a river or lake. But these small amounts of radiation released into the environment are not believed to be harmful, and are actually smaller than the amounts of radioactivity released by fossil fuel burning plants. Thermal pollution remains a problem at some nuclear plants. But cooling towers help correct this problem.
In a small number of nuclear accidents, hazardous amounts of radiation have been released into the atmosphere. Accidental releases of radioactive substances have occurred in Japan, Russia, the United Kingdom, and the United States. An especially serious accident occurred in 1986 at the Chernobyl nuclear power plant in Ukraine (then part of the Soviet Union).
Critics of nuclear power also fear another danger to the environment. As power production increases, the problem of storing high-level radioactive wastes also increases.
Government regulation.
The Nuclear Regulatory Commission (NRC), an agency of the federal government, regulates nonmilitary nuclear power production in the United States. One of the NRC’s main duties is to ensure that nuclear power plants operate safely. Every nuclear reactor and power plant must be inspected and licensed by the NRC before it may begin operations. The NRC also supervises the manufacture and distribution of nuclear fuels, and controls the disposal of radioactive wastes from commercial production.
The Canadian Nuclear Safety Commission, a Canadian government agency, regulates Canada’s nuclear energy industry. The board’s duties resemble those of the Nuclear Regulatory Commission.
Careers in nuclear energy
cover a wide range of occupations and require widely varying amounts of training. A high percentage of the jobs require a college degree or extensive technical education. Many of these jobs are in large research laboratories, which work to improve nuclear processes and to lessen their hazards. Other careers requiring advanced training are in such areas as uranium mining and processing, reactor manufacturing and inspection, power plant operation, and government regulation.
Many colleges and universities offer undergraduate and graduate degrees in such highly specialized fields as nuclear engineering, nuclear physics, and nuclear technology. The industry also employs many workers with college degrees in various branches of engineering and in such fields as biology, chemistry, geology, and medicine. Many vocational and technical schools and some high schools prepare students for specialized jobs in the industry.
The development of nuclear energy
In 1972, scientists discovered that a natural chain reaction had released nuclear energy nearly 2 billion years ago in a uranium deposit in west-central Africa. Two billion years ago, there had been so little radioactive decay that the ore contained enough U-235 for a chain reaction. An accumulation of ground water acted as a moderator to begin the reaction. As heat from the reaction changed the water into steam, less and less water was available to serve as a moderator and the reaction died out. Except for such rare natural occurrences, nuclear energy was not released on a large scale on Earth until 1942. That year, scientists produced the first artificially created chain reaction. Scientific discoveries that have taken place since the late 1800’s have led to the large-scale release of nuclear energy.
Early developments.
Before the late 1800’s, scientists did not suspect that atoms could release nuclear energy. Then in 1896, the French physicist Antoine Henri Becquerel found that uranium constantly gives off energy in the form of invisible rays. He thus became the discoverer of radioactivity. Other scientists soon began experiments to learn more about this mysterious phenomenon.
The beginning of nuclear physics.
In 1898, the great New Zealand-born physicist Ernest Rutherford identified two kinds of radioactive “rays,” which he called alpha rays and beta rays. He and other researchers later showed that these rays are actually high-energy particles, which became known as alpha and beta particles. Experiments with these particles then led Rutherford to discover the atom’s nucleus. This achievement, which Rutherford announced in 1911, marked the beginning of a new science—nuclear physics.
Loading the player...Atomic transmutation
About 1914, scientists began doing experiments to see what happens when nuclear particles collide. The scientists used alpha particles from naturally radioactive materials to bombard the nuclei of light atoms. Light nuclei do not repel positively charged particles, such as alpha particles, as strongly as heavy nuclei do. Rutherford used this method to produce the first artificial transmutations in a series of experiments from 1917 to 1919. He bombarded nitrogen atoms with alpha particles. In rare collisions, a nitrogen 14 nucleus absorbed an alpha particle (a helium 4 nucleus). At the same time, the alpha particle pushed a proton out of the nitrogen nucleus. The nucleus thereby became an oxygen 17 nucleus.
Artificial fission.
To produce nuclear reactions in heavy nuclei, scientists needed a particle that heavy nuclei would not repel. In 1932, the British physicist James Chadwick discovered such a particle—the neutron. In 1938, two German radiochemists, Otto Hahn and Fritz Strassmann, reported they had produced the element barium by bombarding uranium with neutrons.
At first, scientists could not explain how uranium had produced barium, which is much lighter than uranium. All previous transmutations had resulted in an element about as heavy as the original one. Then in 1939, the Austrian physicist Lise Meitner and her nephew Otto Frisch showed that Hahn and Strassmann had in fact produced the first known artificial fission reaction. A uranium nucleus had split into two nearly equal fragments, one of which consisted of a barium nucleus. Two neutrons were also emitted. The other fragment consisted of a nucleus of krypton, a somewhat lighter element than barium. These two nuclei, together with the emitted neutrons, are lighter than a uranium nucleus and a neutron. The reaction had therefore produced more energy than it consumed.
Scientists soon realized that if many uranium nuclei could be made to fission, a tremendous amount of energy would be released. The amount of energy could be calculated from a theory developed by the great German-born physicist Albert Einstein in 1905. The theory shows that matter can change into energy and that matter and energy are related by the equation E = mc-squared (also written E = mc2). This equation states that the energy (E) into which a given amount of matter can change equals the mass (m) of that matter multiplied by the speed of light squared (c2). The speed of light squared is obtained by multiplying the speed of light by itself. Using this equation, scientists determined that the fissioning of 1 pound (0.45 kilogram) of uranium would release as much energy as 8,000 tons (7,300 metric tons) of TNT. Uranium could therefore be used to make a powerful bomb.
The development of nuclear weapons.
World War II broke out in Europe in September 1939. The month before, Einstein had written to U.S. President Franklin D. Roosevelt urging him to commit the United States to develop nuclear energy technology, including an atomic bomb. Einstein had fled to the United States from Germany to escape Nazi persecution. He warned Roosevelt that German scientists might already be working on a nuclear bomb. Roosevelt acted on Einstein’s urging, and early in 1940 scientists received the first funds for uranium research in the United States. The United States entered World War II in 1941. The government then ordered an all-out effort to build an atomic bomb and in 1942 established the top-secret Manhattan Project to achieve this goal (see Manhattan Project).
A group of scientists at the University of Chicago had charge of producing plutonium for the Manhattan Project. The group included such noted physicists as Enrico Fermi, Leo Szilard, and Eugene Wigner, all of whom had been born in Europe and had settled in the United States. Fermi headed the group. Under the scientists’ direction, workers built an atomic pile, or reactor, beneath the stands of the university athletic field. The pile consisted of 50 tons (45 metric tons) of natural uranium oxide and uranium embedded in 500 tons (450 metric tons) of graphite. The graphite served as a moderator. The pile was designed to demonstrate a controlled nuclear chain reaction in the uranium. Cadmium rods controlled the reaction. On Dec. 2, 1942, this reactor produced the first artificial chain reaction.
The success of the University of Chicago project led the U.S. government to build a plutonium-producing plant in Hanford, Washington. The government also built a uranium enrichment plant in Oak Ridge, Tennessee. Plutonium and greatly enriched uranium from these plants were used in the two atomic bombs that the United States dropped on Japan in August 1945.
After World War II, scientists began work on developing a hydrogen bomb. The United States exploded the first hydrogen bomb in 1952 and so achieved the world’s first large-scale thermonuclear reaction. The Soviet Union tested its first atomic bomb in 1949 and its first full-scale hydrogen bomb in 1953. China, France, India, Pakistan, South Africa, and the United Kingdom have also exploded nuclear weapons. For more information on the development of nuclear weapons, see Nuclear weapon.
The first peaceful uses.
While research on nuclear weapons continued, various countries also began experimenting with nuclear reactors. The United States and the Soviet Union had built uranium enrichment plants for nuclear weapons. Both countries therefore started to develop light water reactors, which require enriched uranium fuel. Canada, France, and the United Kingdom worked on reactors moderated by graphite or heavy water. These reactors cost more to build than light water reactors but use natural uranium.
The U.S. Congress set up the Atomic Energy Commission (AEC) in 1946 to direct and control all aspects of nuclear energy development in the United States. In 1954, Congress allowed private industry to take over most aspects of commercial nuclear power development. But the AEC became responsible for regulating the nuclear energy industry. It also kept control in such areas as uranium enrichment and waste disposal.
The United States made the world’s first full-scale use of controlled nuclear energy in 1954. That year, the U.S. Navy launched the first nuclear-powered vessel, the submarine Nautilus. The world’s first full-scale nuclear power plant began operations in 1956 at Calder Hall in northwestern England. In 1957, the first large-scale nuclear plant in the United States opened in Shippingport, Pennsylvania. It supplied electric power to the Pittsburgh area until 1982, when the plant was closed. Canada opened its first full-scale plant in 1962 at Rolphton, Ontario.
The successful start of the nuclear power industry convinced world leaders of the need for international cooperation in the field. In 1957, the United Nations (UN) established the International Atomic Energy Agency to promote the peaceful uses of nuclear energy. See International Atomic Energy Agency; United Nations (Peaceful uses of nuclear energy). Also in 1957, Belgium, France, Italy, Luxembourg, the Netherlands, and West Germany formed the European Atomic Energy Community (Euratom). The organization encourages the development of nuclear power among its member countries. Today, the countries that make up the European Union are all members of Euratom (see European Union (EU)).
The spread of nuclear capability.
During the 1960’s and early 1970’s, a number of countries acquired reactors and used them to start nuclear power development. Progress was also made during this period toward limiting nuclear weapons tests and stopping the spread of nuclear weapons. In 1970, for example, a nuclear nonproliferation treaty went into effect. The treaty prohibits the nuclear powers that have agreed to abide by the document from giving nuclear weapons to nations that do not already have them. The nonproliferation treaty also prohibits nations without nuclear weapons from acquiring them.
But the nonproliferation treaty does not prohibit nations from selling or buying nuclear reactors. A reactor can be used not only for peaceful purposes but also to produce plutonium for nuclear weapons. India used a research reactor for this purpose and in 1974 exploded its first atomic bomb. Canada had supplied the reactor to India with the understanding it would be used for peaceful purposes only. Canada has signed the nonproliferation treaty, but India has not. Critics of India’s action questioned the wisdom of supplying reactors to countries that do not already have them.
Meanwhile, the United States had been greatly increasing its nuclear power capacity. But opposition to nuclear power development also increased in the United States during the late 1960’s and early 1970’s. Critics began to question nearly every aspect of nuclear power production, from the cost of uranium enrichment to the problems of waste disposal.
Many critics of the United States nuclear program charged that the government overlooked various safety risks at nuclear plants to promote nuclear power development. Partly as a result of such criticism, Congress disbanded the Atomic Energy Commission (AEC) in 1974 and divided its functions between two newly formed agencies. The Energy Research and Development Administration (ERDA) took over the AEC’s development programs. The Nuclear Regulatory Commission (NRC) took over its regulatory duties. The NRC, it was believed, could better regulate the industry if it was not also responsible for the industry’s growth and development. In 1977, Congress abolished ERDA and transferred its responsibilities to the newly created Department of Energy. See Energy, Department of; Nuclear Regulatory Commission.
In 1992, the U.S. Congress passed the Energy Policy Act, opening the way for deregulation of the electric power industry. Since then, many nuclear facilities have consolidated. In 2000, the NRC began approving extensions of operating life for existing nuclear plants.
Safety concerns.
There have been a number of accidents at nuclear power plants. Most of them have not been serious. However, in 1957, a fire at the Windscale plutonium production plant in northern England resulted in the release of a large quantity of radioactivity. The British government banned the sale of milk from cows in that part of England for more than a month after the fire.
In the United States, concerns about the safety of nuclear reactors increased after a serious accident in 1979 at the Three Mile Island nuclear power plant near Harrisburg, Pennsylvania. Mechanical and human failures resulted in a breakdown of the reactor’s cooling system and serious damage to the reactor core. Scientists and technicians prevented a failure of the reactor vessel that might have released large amounts of radioactive isotopes into the reactor containment building. Cleanup of the plant was completed in the early 1990’s.
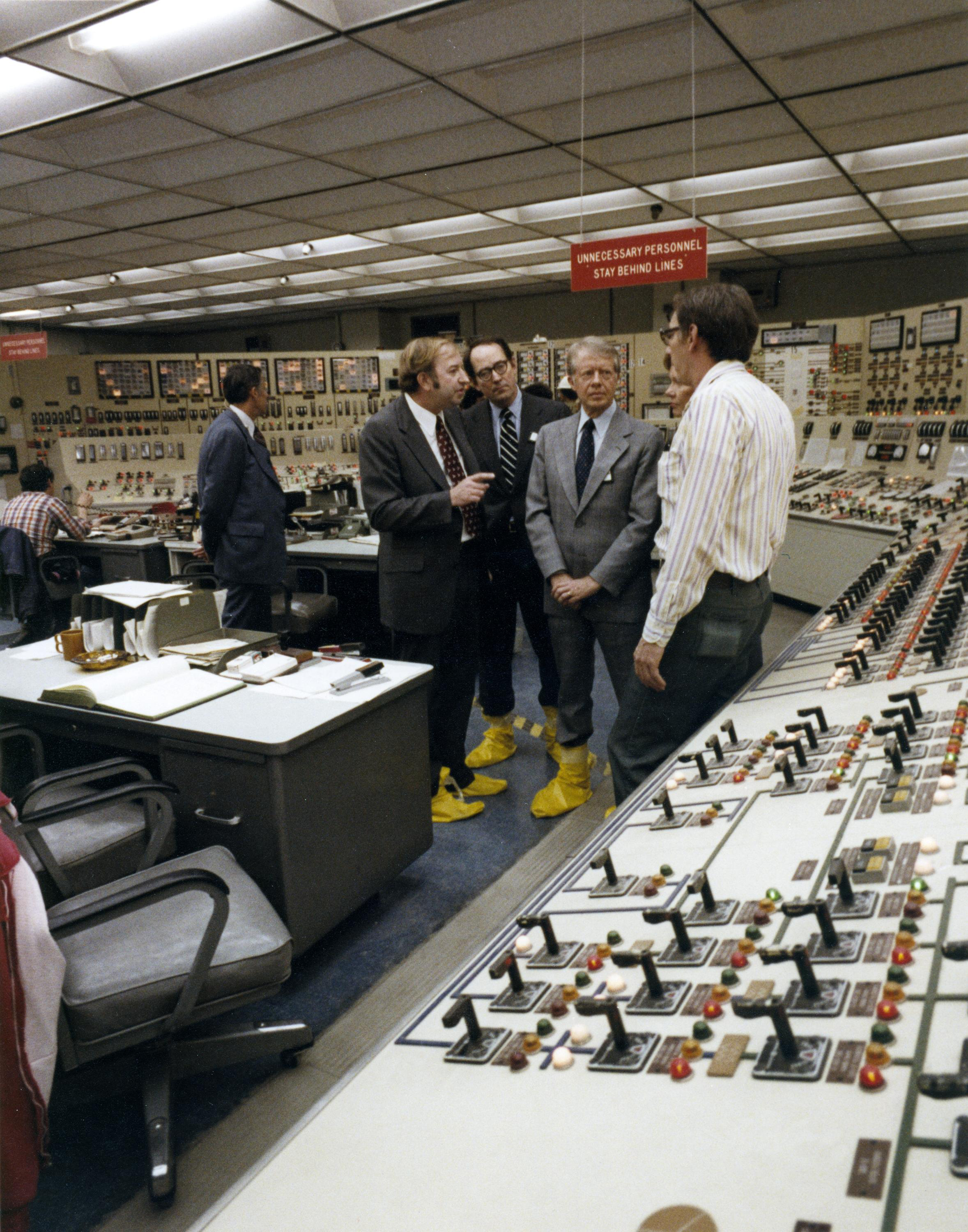
The worst nuclear accident in history occurred in 1986 at the nuclear power plant at Chernobyl (now Chornobyl) near Kyiv in Ukraine, which was then part of the Soviet Union. An explosion and fire ripped apart the reactor and released large amounts of radioactive isotopes into the atmosphere. Unlike most Western reactors, the Chernobyl reactors had a different type of enclosure that was unable to prevent radioactive isotopes from escaping. Soviet officials reported that 31 people died from radiation sickness or burns and more than 200 others were seriously injured. The radioactive substances spread over parts of what are now Ukraine, Russia, and Belarus, and were carried by wind into northern and central Europe. Experts expected a significant increase in the number of cancer deaths among those near the reactor. But they predicted that the health effects outside the area would be slight. The last working reactor at Chernobyl was shut down in 2000.
As a result of the accidents at Three Mile Island and Chernobyl, opposition to nuclear power increased in many countries during the late 1980’s. In the United States, the NRC tightened its control of nuclear plants.
Experts have expressed particular concern over the safety of older Soviet-designed reactors now operating in Russia, Ukraine, and several countries of the former Soviet bloc. Western scientists and engineers are helping to remedy some of the most urgent safety problems in these reactors. Most have been closed or redesigned to eliminate their original design flaws.
Research on new types of reactors.
New research on reactors was sparked by fears of a shortage of U-235 to fuel fission reactors. However, during the 1970’s nuclear power production increased less rapidly than expected. Geologists also discovered that there was much more uranium on Earth than had originally been estimated. Therefore, the expected near-term shortage did not occur.
Today, scientists seek to develop safer and more efficient reactors. Researchers have concentrated their efforts on the development of a fusion reactor and advanced fission reactors.
Experimental fusion devices.
Most experiments on controlled nuclear fusion have concentrated on the development of reactors that would use deuterium and tritium plasma as the fuel. One main fusion reactor design, called a tokamak, was originally developed by Soviet scientists. The word tokamak comes from Russian words meaning toroidal (doughnut-shaped) chamber and magnetic coil. Like many other experimental fusion reactors, a tokamak uses magnetic confinement. A magnetic field pushes plasma away from the tokamak’s containing walls. The tokamak also passes a strong electric current through the plasma. The current interacts with the magnetic field to help confine the plasma.
Another kind of fusion reactor uses inertial confinement. In this case, no container holds the fuel during fusion. Rather, the fuel’s inertia, its natural tendency to remain in the same place, holds it long enough for fusion to occur. In one kind of inertial confinement fusion (ICF) reactor, powerful laser beams strike a cylindrical case made of metal. Inside the case is a pea-sized sphere containing deuterium and tritium gas. The laser beams heat the case, causing it to turn to plasma. The plasma, in turn, bombards the sphere with X rays, rapidly heating the shell of the sphere. As a result, the shell both expands and pushes inward. The inward push compresses the fuel, causing fusion.
The most powerful laser-driven reactor is operated by the National Ignition Facility (NIF) at Lawrence Livermore National Laboratory in Livermore, California. The facility uses 192 separate lasers to deliver a powerful pulse of energy lasting a tiny fraction of a second.
A major goal of fusion research is to reach the breakeven level, the level of energy production equal to the energy needed to create and heat the plasma. This goal was achieved for the first time ever in an experiment at NIF on Dec. 5, 2022. The fusion reaction produced 1 1/2 times the amount of energy delivered by the lasers to create the reaction.
Overall, however, current laser-driven reactors are inefficient. To create a fusion reaction, the lasers transfer an immense amount of energy to the fuel. But hundreds of times as much energy is needed to generate the lasers. Therefore, even if an experiment reaches the breakeven level, there is still a huge overall loss of energy. Because of this inefficiency, many researchers think a tokamak design is more practical for producing electricity.
For a tokamak of current design to reach the breakeven level, it must heat the plasma to at least 180,000,000 °F (100,000,000 °C). Also, each cubic centimeter (0.061 cubic inch) of the plasma must contain about 100 trillion nuclei, and the magnetic field must confine the plasma for about 1 second.
Researchers working with tokamaks have reached these three goals, but never in the same experiment. Scientists hope that ITER, an experimental nuclear fusion reactor, will reach or exceed the breakeven level in the future.
Advanced fission reactors.
Research, development, and design work on advanced fission reactors has concentrated on advanced versions of today’s light-water reactors, other types of advanced thermal reactors, and fast reactors. The goals of this work include improved operating efficiency, simplified design, lower cost, and increased use of passive safety systems. A passive safety system is one that does not depend upon mechanical devices, such as pumps and valves operated by people or powered by electricity, to prevent core damage and remove heat from the core if an accident occurs.
A type of fission reactor that is currently operating in Japan and France—and which the NRC has certified for use in the United States—is the advanced light water reactor (ALWR). ALWR’s have many passive safety features. One ALWR design relies on pools of water positioned at various heights. Gravity, rather than pumps, circulates a supply of cooling water that would be needed in certain emergencies.
A high-temperature gas-cooled reactor (HTGR) is a smaller power plant that uses a pressurized gas, usually helium, as the coolant. The fuel consists of ceramic-coated pellets of uranium oxy-carbide. The purpose of this design is to make it extremely unlikely for the fissions in a pellet to produce enough heat to rupture the casing, even if the reactor were to lose all its coolant. If the pellets remained intact, radioactive material would not be released.
A group of reactors known as fast reactors have long-term advantages. A fast breeder reactor can be designed to both consume plutonium as a fuel and convert U-238 to more plutonium. The plutonium, in turn, can be used as reactor fuel. The breeder thus creates more fissionable material than it consumes. Because uranium is plentiful, the use of breeders would extend its potential as an energy resource enormously. Several countries have built experimental breeders. A unit in France, called the Superphenix, produced 1.2 million kilowatts of electric power.
Fast reactors can also be developed to consume some of the long-lived radioactive materials, such as plutonium and americium, which are in spent nuclear fuel from light water reactors. They thus avoided the need to contain these materials in a repository for thousands of years.