Cell is the basic unit of all life. All organisms (living things) are made up of one or more cells. Some organisms, such as many bacteria, consist of only one cell. Most plants and animals have many cells. The human body has more than 10 trillion (10,000,000,000,000) cells.
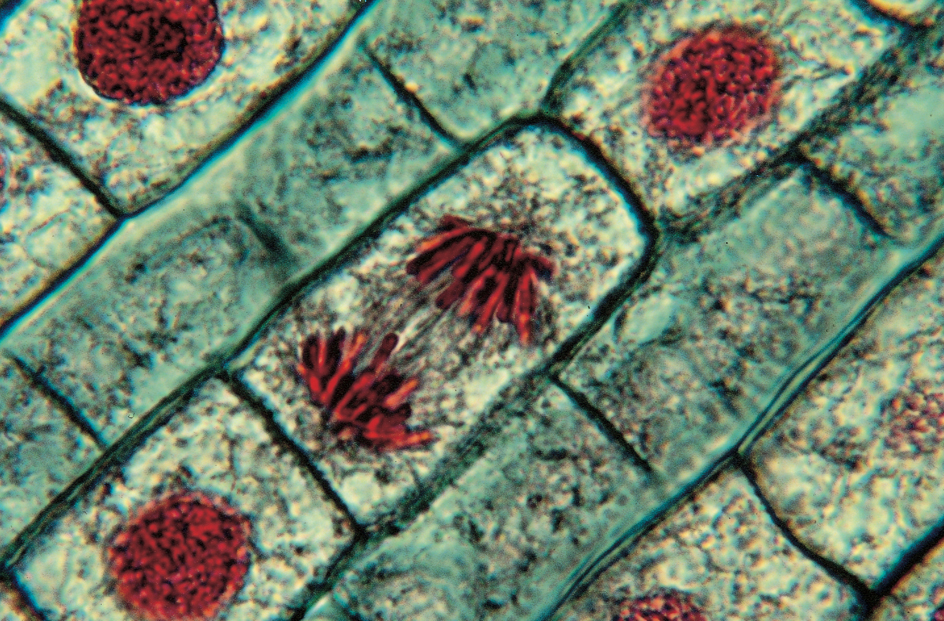
Most cells are so small they can be seen only with a microscope. It would take about 40,000 of your red blood cells to fill this letter O. It takes millions of cells to make up the skin on the palm of your hand.
Some organisms spend their entire lives as a single cell. Other organisms live only as collections of cells. Nearly all the cells of plants and animals have specialized jobs. As you read these words, for example, nerve cells in your eyes are carrying signals about what you are seeing to your brain cells. Your brain cells, in turn, may send signals back to tell your muscle cells to cause some action, like moving your fingers or opening your mouth to say something. Groups of cells that work together form the different tissues of the body, such as skin, nerve, and muscle tissue. Different kinds of tissues, in turn, can work together to form organs, such as the brain, eyes, heart, and lungs. Together, all the specialized cells, tissues, and organs form you—or some other complete organism.
All cells have some things in common, whether they are single-celled organisms or specialized cells within a body. A cell is alive. Cells “breathe,” take in food, and get rid of wastes. Cells also grow and reproduce (create more of their own kind). And in time, cells die.
All cells have a covering called a membrane. The membrane encloses the cell, separating the cell’s contents from its surroundings. The contents inside the cell membrane are called the cytoplasm.
Many cells have a structure called the nucleus. The nucleus holds most of the cell’s hereditary material. This material provides the genetic instructions that control almost everything the cell does. Other cells do not keep their hereditary material inside a nucleus.
Just as all living things are made up of cells, every new cell is produced from an existing cell. Cells reproduce by dividing, so that at the end of the division process, there are two cells where there once was only one. Each of the newly produced cells has a copy of the genetic material that was in the original cell.
The genetic material is a chemical substance called DNA (deoxyribonucleic acid). All DNA consists of the same building blocks, but the order and number of these blocks can be different in every organism. This variation is a major part of what makes you different from other people. It is also what makes a dog different from a fish, a zebra different from a rose, and a willow different from a wasp.
DNA represents a set of instructions that guides the activities of the cell. The instructions take the form of a chemical code that determines, among other things, what the cell makes, what it needs, and when it should divide. When something goes wrong with a cell, leading to a disease such as cancer, scientists may directly analyze these chemical instructions to discover the problem. In some cases, scientists can correct errors in the cell’s instructions. By altering a cell’s genetic instructions, it is also possible to create genetically modified organisms (GMO’s) with new characteristics. The new traits of such organisms can have commercially important applications. For example, scientists have developed varieties of rice that produce beta carotene, an ingredient that enables the body to produce vitamin A. Having enough vitamin A in the diet can help prevent certain forms of blindness.
Looking at a cell
One of the most important tools scientists use to study cells is the microscope. A traditional microscope, called an optical microscope, can magnify a cell up to 2,000 times. When scientists use this type of microscope, they may add certain dyes to make various parts of the cell stand out clearly. However, the details of some parts still cannot be seen. A type of microscope called an electron microscope can magnify a cell by 1 million times. Advances in computers and engineering have enabled researchers to create new, special types of microscopes to produce images of ever smaller objects within cells. Some of these devices can image individual molecules in certain cases.
Another tool used to study cells is the centrifuge. To use this tool, scientists first grind up some cellular material and put it into a tube. The tube is placed in a centrifuge and whirled around rapidly to separate the cellular parts. The heaviest parts move to the bottom of the tube, and the lightest remain at the top. After the parts have been separated, scientists can collect the different parts to study their chemical content and activity.
Shapes of cells.
Cells come in a tremendous variety of shapes and sizes. Cells can be shaped like boxes, coils, corkscrews, cubes, octopuses, rods, saucers, stars, tubes, or blobs of jelly. Many unicellular (one-celled) organisms, including some yeasts and certain bacteria, look like tiny balls or rods. The ameba, another unicellular organism, looks like a flattened jellylike mass that constantly changes its shape to move about. The ocean is filled with diatoms, one-celled algae that come in a wide range of shapes, including cubes, pyramids, and spheres.
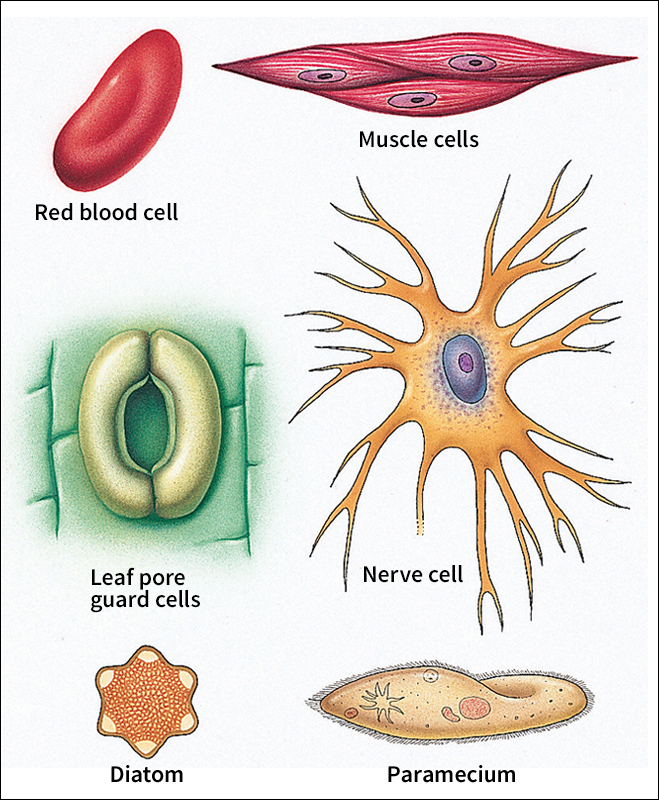
Many of the cells of multicellular (many-celled) plants are shaped like cubes or many-sided boxes. The greatest variety in cell shapes occurs in human beings and other multicellular animals. A cell’s shape is related to its needs or to the job it does. Some muscle cells are long and thin. This enables them to contract or expand, moving the arms and legs. Some nerve cells have multiple long branches, somewhat like the branches of a tree. The branches of one nerve cell can connect to others, relaying signals throughout the body. Inside the intestines, some individual cells have fingerlike projections. These cells have a large surface area for their size, which helps them to absorb nutrients and other molecules from food.
Sizes of cells.
Cells vary widely in size. Most cells are about 1/1,000 of an inch (0.0025 centimeter) in diameter. About 500 to 1,000 of these average-sized cells would fit within the period at the end of this sentence.
Bacterial cells are among the smallest of all cells. Certain kinds of bacterial cells are so small that a row of 50,000 of them would measure only 1 inch (2.5 centimeters) long. Some animal cells are relatively large. Bird eggs, for example, are single cells when they are laid. One of the largest of all cells is an ostrich egg.
The size of an organism usually depends more on the total number of cells it has and not as much on the size of the cells. An elephant is a giant compared with an ant because the elephant has trillions more cells.
Inside a living cell
Although cells differ greatly in size and shape and in the special jobs they do, most cells have certain features in common. Generally, a cell can be thought of as a tiny chemical factory, with a control center that tells it what to do and when. Almost all cells have structures called ribosomes in which proteins are manufactured. These proteins make it possible for the cell to grow, repair itself, and perform thousands of chemical operations required during its lifetime. Many cells also have structures that act as “power plants,” generating the energy needed to function. In multicellular organisms, a cell may have special equipment to fulfill its role within the body.
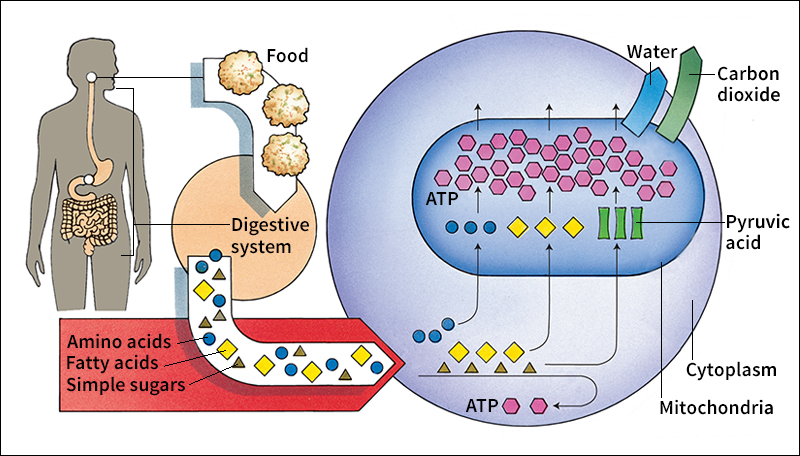
The cell membrane, sometimes called the plasma membrane, is a thin layer that encloses the cell and helps control the passage of substances in or out. It con sists of a double layer of a fatty material called phospholipid. Some cells have an additional special covering that helps protect them or hold them to neighboring cells. In plant cells, this covering is called the cell wall.
Animals and plants are classified as eukaryotes because most of their cells have a true nucleus. In these organisms, only a few cells, for example red blood cells, lack a nucleus. The eukaryotes also include fungi and such unicellular organisms as amebas and diatoms. Archaea and bacteria cells lack a nucleus. They are called prokaryotes, which means before the nucleus.
The nucleus
is the control center that directs the activities of the cell. A nuclear membrane surrounds the nucleus and separates it from the cytoplasm. The nucleus contains two important types of structures, chromosomes and nucleoli.
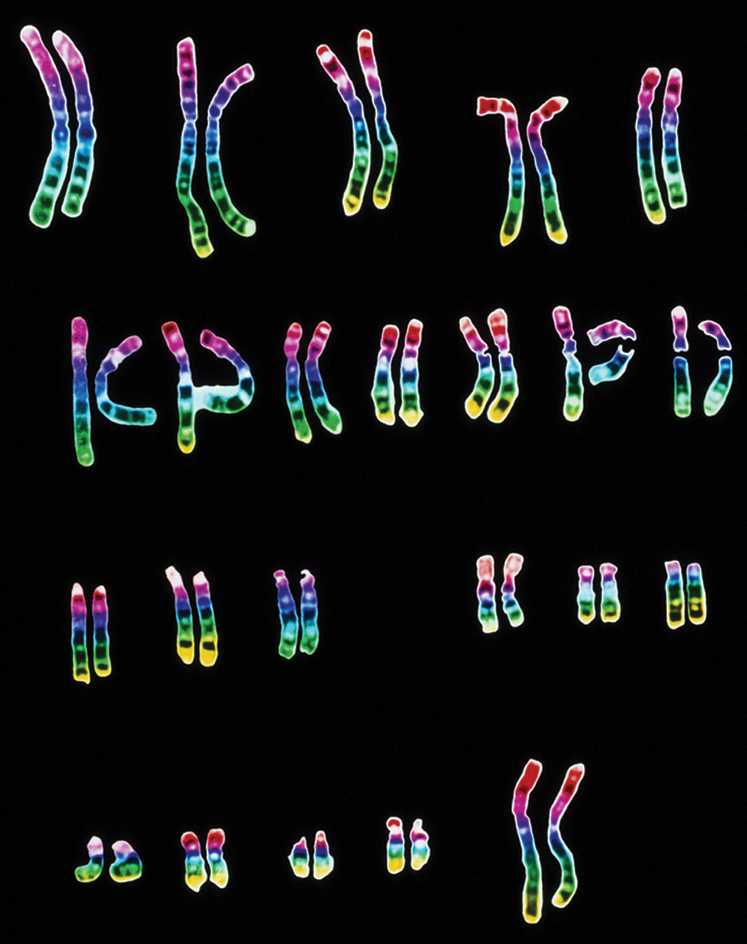
Chromosomes are made up of long, threadlike strands of a substance called chromatin. Chromatin consists of DNA and certain proteins. This DNA includes the genes, the basic units of heredity. The DNA within genes determines many of a living thing’s features, such as a person’s blood type, the color of the eyes, the texture of the hair, and thousands of other characteristics. Genes are passed from parents to their offspring.
DNA works by presenting a set of instructions for building proteins. These proteins are needed to make many of the structures found in cells. They include molecules called enzymes that speed up chemical reactions. Without enzymes, the cell could not function normally. Thus, the kinds of proteins a cell makes help determine the nature of the cell.
Nucleoli (singular, nucleolus) are round structures that help in the formation of ribosomes, the centers of protein production. Each nucleus may contain one or more nucleoli, though some cells have none. Nucleoli are made up of proteins and RNA (ribonucleic acid). RNA is chemically similar to DNA and plays an important role in making proteins.
The cytoplasm
is all the material enclosed by the cell membrane, except for the nucleus if the cell has one. Many cells contain small structures in the cytoplasm called organelles. Each organelle has a particular job to do. The organelles include the nucleus, mitochondria, endoplasmic reticulum, and Golgi complex. Some cells have other organelles, such as lysosomes, vacuoles, or chloroplasts. All eukaryotic cells also contain a network of proteins known as the cytoskeleton.
Mitochondria are the “power plants” of the cell. A cell may contain hundreds or even thousands of mitochondria. These structures convert the chemical energy from food into a form the cell can use to grow, divide, and do its work.
The endoplasmic reticulum is a complex network of membranes. This network forms a system of pouches sandwiched between the nucleus and the Golgi complex. Some parts of the endoplasmic reticulum have a smooth surface. Other parts of the membrane have a rougher appearance because of the many ribosomes attached to their surface. Many of the cell’s proteins are made in the endoplasmic reticulum.
The Golgi complex, also known as the Golgi apparatus, consists of a stack of flat membrane sacs. These sacs process proteins and other substances produced in the cell. Small spheres called vesicles pinch off from the Golgi complex and move some of these substances to the cell membrane. The substances then may be transported across the membrane to other cells in the body o r used to make new membrane material. Other Golgi vesicles remain inside the cell and fuse with each other to form compartments that store proteins or other substances.
Lysosomes are round bodies containing enzymes that can break down many substances. For example, lysosomes inside white blood cells can destroy harmful bacteria. In plant cells and certain unicellular organisms, large, fluid-filled vacuoles usually perform the same function as lysosomes. In some plant cells, a single vacuole can take up most of the space in the cytoplasm.
Chloroplasts are organelles found in the cells of plants and algae. They bear many similarities to mitochondria. The chloroplasts contain a green substance called chlorophyll . Chlorophyll captures the energy of sunlight. Chloroplasts use this energy to make sugars that are rich in chemical energy, in a process called photosynthesis. Nearly all living things directly or indirectly depend on these sugars for the energy to make all the other chemical substances in cells. For example, many animals get energy by eating plants or by eating animals that have eaten plants.
The cytoskeleton consists of several types of proteins that form a complicated network of rods and support structures in the cytoplasm. The cytoskeleton gives a cell its overall shape, and it keeps organelles in the cell in their proper positions. In some cases, the cytoskeleton enables the cell to move. Cells that move by swimming do so by means of hairlike structures that extend out from the cell. These structures include cilia and flagella, both of which contain bundles of flexible cytoskeletal rods. In many cells, some of the cytoskeleton is found in the centrioles, a pair of short, cylinder-shaped structures involved in cell reproduction. Centrioles lie at right angles to each other, usually near the nucleus.
Inside bacteria.
Bacteria are extremely small, single-celled organisms that lack a true nucleus. But they have DNA. In bacteria, the DNA is normally in the form of a ring-shaped molecule. The region inside the bacterial cell that contains DNA is called the nucleoid. Bacteria do not have the same cytoskeletal structures or any of the membrane-surrounded organelles found in eukaryotic cells. Some bacteria have flagella, but they differ in structure from those of eukaryotic cells. These flagella may rotate like the blades of a propeller to make the bacteria move.
The life of a cell
Every living thing is made up of one or more cells, and each of these cells is the offspring of an existing cell. New cells are formed by division, so that there are two cells where there once was only one cell. In multicellular eukaryotes, each of the two new cells produced by division is often called a daughter cell. One-celled organisms, such as bacteria, will grow and divide, but each resulting cell remains a separate living thing.
Human beings and other multicellular organisms develop from a single cell called a zygote. When the zygote grows to a certain size, it divides and forms two cells that remain attached to each other. These two cells grow and divide again, forming four cells. These cells continue to grow and divide over and over again, eventually packing themselves into a tight ball. From this point, as the cells continue to divide, they begin to take on specialized shapes and jobs, eventually forming all the different parts of the body.
Cell division
involves two processes. In the first process, called nuclear division, the nucleus divides. In the second process, called cytokinesis, the cytoplasm divides, and the cell splits in half. There are two types of cell division in eukaryotes: (1) mitosis and (2) meiosis.
Loading the player...Cell division
Mitosis.
Most eukaryotic cells divide by mitosis. Mitosis is a continuous process, but it can be described in terms of four basic stages. These are: (1) prophase, (2) metaphase, (3) anaphase, and (4) telophase.
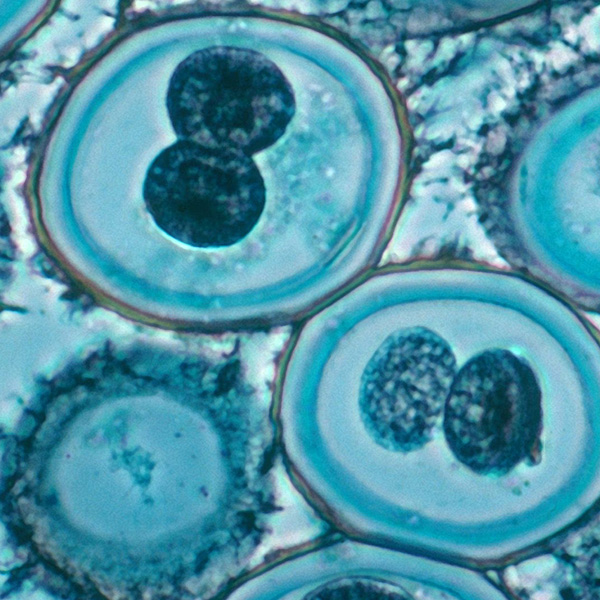
In prophase, the cell’s chromosomes begin to coil up and condense into visible threads that become progressively shorter and thicker. As the chromosomes condense, part of the cytoskeleton organizes into a network of fibers called spindles that extend across the cell. The centrioles move apart along the spindle fibers until they are at opposite sides of the cell. The centrioles mark the poles of the spindle. Toward the end of prophase, the nuclear membrane breaks apart.
In metaphase, the second stage, the chromosomes move to the middle of the spindles, called the equator. Each chromosome at this point consists of two attached, identical strands, called sister chromatids. Each sister chromatid is attached to its partner by a structure called a centromere. The chromosomes line up along the cell’s equator, with each sister chromatid attached at the centromere to at least one spindle fiber. In the third stage, called anaphase, the centromeres divide, and each sister chromatid becomes a separate chromosome. The new chromosomes separate and move to opposite ends or poles of the cell.
In telophase, the end stage of mitosis, individual chromosomes uncoil and again become hard to see. A new nuclear membrane forms around each new daughter nucleus. Also, the spindle breaks down, and the proteins from spindle fibers form part of the networks of cytoskeleton in the daughter cells.
Usually, division of the cytoplasm also begins during telophase. In animal cells, cytokinesis occurs when the cell membrane pinches between the two nuclei to form two daughter cells. In plant cells and other cells that have a cell wall, a cell wall also grows between the two nuclei, forming two cells. In either case, each new cell has as many chromosomes as the original cell and contains the same hereditary information.
Cytokinesis does not always create two identical cells. Sometimes, one of the daughter cells receives more of one kind of organelle than does the other cell. Cytokinesis may also result in two different sized cells. In addition, nuclear division may occur more than once in the same cell without cytokinesis, resulting in cells with more than one nucleus.
Meiosis
is a specialized form of cell division that occurs in certain cells of human beings and many other living things that reproduce sexually. Among such organisms, a new individual begins when a male sex cell, called a sperm, unites with a female sex cell, called an egg, to form a zygote. The sex cells that produce eggs or sperm are also called germ cells, and they are produced in special reproductive tissues or organs. At first, the germ cells grow and divide by mitosis like other cells. To produce the sperm or egg cells, however, they must go through meiosis. To understand why, we must understand something about heredity.
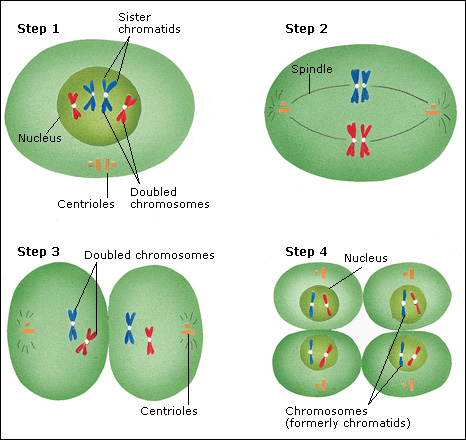
Every species of life has a certain number of chromosomes in each of its somatic (body) cells. These chromosomes exist in pairs. For example, human beings have 23 pairs of chromosomes; frogs, 13 pairs; and pea plants, 7 pairs. The members of each pair are usually similar in size, shape, and hereditary content.
In human beings, for example, each somatic cell has 46 chromosomes. If the father’s sperm cells and the mother’s egg cells each contained 46 chromosomes, they would unite to form a zygote with 92 chromosomes—double the correct number. To prevent this, the process of meiosis produces sperm or egg cells that each have only half the number of chromosomes found in the somatic cells. When these unite, the correct total number is restored in the zygote.
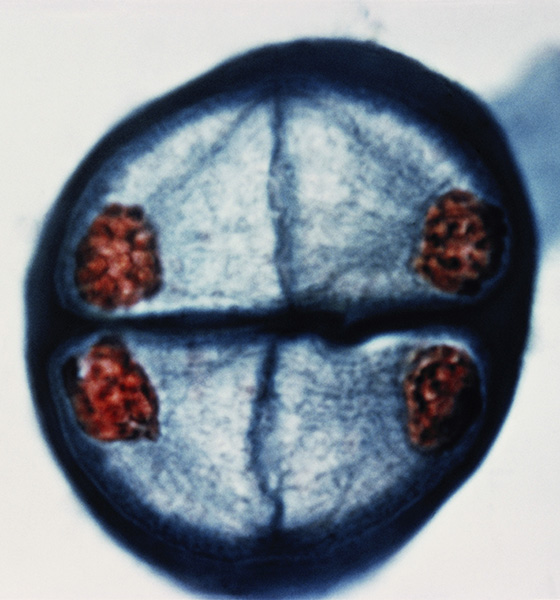
Meiosis consists of two separate nuclear divisions. In the first part of meiosis, each duplicated chromosome lines up side by side with the other chromosome of its pair, positioned on opposite sides of the equator. The pairs are then separated, with one chromosome of each pair going to one pole, while the other chromosome moves to the opposite pole. Cytokinesis occurs, dividing the cytoplasm in two. Each daughter cell thus receives one chromosome from each of the original pairs. This division is the key division of meiosis, reducing the total number of chromosomes in each cell to half of the original number.
Each of the new cells then divides again. In this second division, which more closely resembles mitosis, the chromosomes divide, with one of each pair of sister chromatids going to each new daughter cell. Cytokinesis occurs again, and two daughter cells are formed from each division. Each complete round of meiosis thus produces a total of four cells. Each of these cells, whether a sperm or an egg, contains half the number of chromosomes found in the other cells of the organism. When sperm and egg are later combined, in the process called fertilization, they produce a zygote with the correct total number of chromosomes.
The cell cycle.
Cell division is part of a larger process known as the cell cycle. A cell must complete this cycle each time it undergoes mitosis. The cell cycle has four different phases, designated G1, S, G2, and M. The M phase—M stands for mitosis—includes the division of the nucleus and the cell itself. Before the cell can enter the M phase, however, it must go through the G1, S, and G2 phases to prepare for division. These preparatory phases are together referred to as interphase.
G stands for gap. In the G1 phase, the cell is preparing all of the enzymes and other ingredients that it will need to copy its genetic material. In the S phase—which stands for synthesis—the cell makes or synthesizes a copy of the genetic material. The genetic material is organized into chromosomes, but at this stage, they are not visible using an optical microscope. During the S phase, each chromosome is copied to produce a pair of sister chromatids joined by a centromere. After the S phase is complete, the cell enters a second gap phase, G2, in which new copies of organelles and other parts of the cell are made. When interphase is finished, the cell is ready for the M phase to complete the cell cycle.
Growth and specialization
are the processes that guide how a zygote develops into an organism. After a large mass of cells is formed, the cells begin to differentiate (specialize), becoming muscle cells, skin cells, nerve cells, and so on. The specialized cells also group into tissues. These tissues then form organs, such as the heart and lungs.
Understanding differentiation is a challenge for scientists. Every time a cell divides, it passes on the same hereditary material. Scientists think that differentiation occurs when a specific set of genes becomes active in a cell. These genes produce certain proteins, many of which are enzymes or other specialized proteins that cause the cell to differentiate into a particular cell type. Much remains to be learned about how only certain genes are activated in each type of cell.
Death of a cell.
Like all other living things, cells die. Each day, several billion cells in the body die and are replaced through cell division. Dead skin cells flake off. Dead cells from internal organs pass out of the body with waste products.
The life span of cells varies. For example, most white blood cells live about 10 hours, but some white blood cells live about 13 days; red blood cells live about 120 days; and liver cells live about 10 months. Most nerve cells can live for many years, often for the lifetime of the organism.
The work of a cell
A cell is intensely active. Cells carry out life’s functions, including growth and reproduction. In addition, cells in multicellular organisms have special jobs. To live and to do its work, a cell must obtain energy. It also must manufacture proteins and other substances needed for the construction of its parts and to speed up the thousands of chemical reactions that occur in the cell.
Producing energy.
Most of a body’s energy comes from the mitochondria, the power producers of a cell. The mitochondria can be compared to power plants. Power plants use fuel to produce the electric power that runs machines. Likewise, the food a person eats provides the fuel that is “burned” inside the mitochondria. A product of this burning is a compound called adenosine triphosphate (ATP). ATP is the power source that runs a cell’s activities. It supplies the energy needed to do work in the cells. For example, ATP supplies the energy to contract a muscle or send a message between nerve cells.
An ATP molecule contains three chemical structures called phosphate groups. Chemical bonds link the phosphate groups together like railroad cars. The bonds that attach the second and third phosphate groups are especially rich in energy. When the bonds are broken, energy is released that the cell can use.
The ultimate source of energy for most living things—directly or indirectly—is the sun. Cells of plants, algae, certain bacteria, and some other living things capture energy from the sun and use it to make sugars via photosynthesis. Many leaves appear green as a result of chlorophyll molecules in their chloroplasts. The chlorophyll absorbs energy from the sun, setting off a series of chemical reactions. ATP is produced, and this, in turn, provides the energy that a plant uses to combine carbon dioxide from the air with water to make sugars and other substances.
Animal cells obtain their energy from food that the animal eats. The animal’s digestive system breaks down the food into basic parts. It breaks fats into fatty acids, sugars and starches into simple sugars, and proteins into chemical units called amino acids. The blood carries these substances to cells in the body.
In the cell’s cytoplasm, the simple sugars are broken down into a chemical called pyruvic acid, and a small amount of ATP is produced. The broken down materials then enter the mitochondria. Enzymes in the mitochondria break down these substances further in a series of chemical reactions. Oxygen must also be present in the mitochondria for these reactions to take place. The reactions produce carbon dioxide, water, and many molecules of ATP. The ATP molecules from the mitochondria provide energy wherever it is needed in the cell. For every job that requires energy, special enzymes break the ATP phosphate bonds and release the energy.
Producing proteins.
All living things contain proteins. The structures of a cell are built largely of proteins. Enzymes, most of which are proteins, speed up the chemical reactions of life. Enzymes help digest food and produce energy and assist in building other proteins. A single cell may contain hundreds of different kinds of enzymes. Many hormones, the substances that regulate chemical activities throughout the body, are proteins. The body also makes proteins called antibodies to fight disease germs.
Proteins are complex, three-dimensional substances composed of one or more long, folded chains of amino acid units. These chains are also called polypeptide chains. There are 20 kinds of amino acids commonly involved in protein production, and any number of them may be linked in any order to form a polypeptide chain. Some polypeptide chains may contain only 10 amino acid “links,” whereas other chains contain hundreds or more. Each different arrangement of amino acids forms a different polypeptide chain. The number of different chains—and thus, different proteins—that can be formed is practically unlimited
DNA contains instructions for making all the proteins found in a cell. DNA directs the number and order in which amino acids will be linked together to form proteins. Protein manufacture takes place in the cytoplasm of the cell. However, the DNA does not leave the nucleus to help make the proteins. This job is done by DNA’s chemical “cousin,” RNA. RNA is made in the nucleus of the cell. Once it is made, it is usually passed out of the nucleus into the cytoplasm.
To understand how proteins are made, let us trace the production of a protein that consists of one polypeptide chain. The first step takes place in the nucleus. There, the DNA guides the production of RNA. The RNA then leaves the nucleus and enters the cytoplasm. This RNA, called messenger RNA (mRNA), goes to the ribosomes, the cell’s centers of protein production. In the ribosome, the information coded on the messenger RNA is “read.” The ribosome constructs the protein by assembling the amino acids in the exact order called for by the DNA of the genes.
Another type of RNA, called transfer RNA, is responsible for bringing each correct amino acid into the ribosome as the mRNA passes through. There are specific transfer RNA molecules for each kind of amino acid. The specific transfer RNA and the correct amino acid are brought together with the help of ATP and an enzyme. One by one, the amino acids are linked together to form the polypeptide chain. Even before the construction of the protein is finished, the chain begins to fold up in precise ways determined by the amino acids it carries.
In most proteins with more than one polypeptide chain, the chains are manufactured separately and then combined to make the complete protein. The finished protein then starts to do its particular job. Some proteins are used inside the cell. Other proteins, such as hormones and digestive enzymes, are released from the cell to do their work.
The genetic code
DNA controls the life of the cell—and the lives of organisms made up of cells—in two ways. First, DNA determines the form and function of the cell by determining when and where different proteins are produced. Second, DNA carries the hereditary information that is passed from one generation of cells to the next and from parents to children. Thus, DNA serves as the master plan of all life.
The DNA molecule
is found in the chromosomes of a cell. Each chromosome probably contains one extremely long DNA molecule. On average, a single human chromosome consists of a DNA molecule almost 2 inches (5 centimeters) long. But the DNA molecule is a thread so thin that only some of its details can be seen when magnified by an electron microscope. Scientists have determined the structure of the DNA molecule primarily on the basis of its chemical composition. They have determined the molecule’s shape by bouncing X rays off the atoms in the molecule and then studying the patterns the scattered X rays made on photographic plates. The patterns show that the molecule normally has the shape of a ladder that is coiled like a spring. This shape is called a double helix. All DNA molecules have this shape.
The DNA ladder includes four building blocks called nucleotides. In DNA, each nucleotide consists of a sugar called deoxyribose joined to a phosphate and one of four compounds called bases. The bases are adenine, cytosine, guanine, and thymine (abbreviated A, C, G, and T). The sides of the ladder contain alternating units of phosphate and sugar. The rungs are made up of bases. Each rung consists of a pair of bases: A-T, T-A, C-G, or G-C. No other combination is possible, because only the A-T and C-G pairs are chemically attracted and only these pairs make rungs of the proper length to fit between the ladder’s side pieces.
This restriction also means that the order of the bases in one half of the ladder, or strand, determines the order of the bases in the other strand. For example, if a sequence of bases in one strand is ATCGAT, the sequence in the corresponding section of the opposite strand must be TAGCTA.
Before a cell divides, the DNA has to be duplicated, or copied. To accomplish this, the ladder is split lengthwise, separating the two bases that make up each rung. Then each half ladder pairs up with unattached nucleotides. The bases in each half ladder can pair up only with their complementary bases. The A’s attach to T’s, the T’s to A’s, the G’s to C’s, and the C’s to G’s. In this way, each new ladder becomes a duplicate of the original ladder. These duplicate DNA molecules can be seen at mitosis as the two sister chromatids of a chromosome. When the cell undergoes mitosis and cytokinesis, each new daughter cell receives identical DNA molecules.
The RNA molecule
carries the DNA’s instructions for protein production. It resembles DNA in many ways. But there are two major differences. The sugar in RNA is ribose instead of deoxyribose, and RNA contains the base uracil (abbreviated U) instead of thymine. Like thymine, uracil will pair only with the base adenine. RNA’s other three bases—A, C, and G—and the phosphate unit are identical to those in DNA.
To make a protein, messenger RNA must first copy the ”blueprints” encoded in DNA. First, a part of the DNA molecule unwinds, and the two strands separate, exposing bases on the ladder’s interior. One of the strands then serves as a template for lining up RNA nucleotides. The bases of unattached RNA nucleotides pair up one at a time with the exposed DNA bases to form the messenger RNA. For example, the RNA bases AUCGAU will pair up with the DNA bases TAGCTA. The completed strand of messenger RNA, which may consist of hundreds of bases, separates from the DNA template and carries the instructions for making a protein out of the nucleus to the ribosomes. The bases of the DNA molecule rejoin and the ladder winds once again, “storing” the chemical instructions.
Decoding genes.
The genetic code lies in the order of the bases in the DNA molecule. This order of bases is passed on from one generation of cells to the next and from one generation of an organism to the next. It is this code that determines that an elephant gives birth to an elephant, not a zebra. It is this order that determines the color of your eyes, the shape of your ears, and thousands of other traits.
The order of the bases in a gene’s DNA determines the order of the bases in the messenger RNA. The messenger RNA in turn determines the order and composition of the amino acids in a specific protein. Thus, the instructions for making a particular protein lie in the chemical structure of a specific gene.
Four different bases are contained in DNA or RNA. But these bases must code for 20 different amino acids commonly used to make proteins. DNA overcomes this problem by storing chemical instructions in a triplet code. In this system, a group of three bases forms the codon for a specific amino acid. Each codon can be de scribed using the order of its bases. For example, the codon UUU consists of three uracil bases in a row.
Scientists have decoded the genetic code. The first codon to be solved was the codon UUU in RNA. Scientists used an RNA chain consisting of only the base uracil repeated over and over again. They added this RNA to a mixture containing the 20 amino acids and the cell’s protein-making machinery. The RNA produced a protein chain consisting of only the amino acid phenylalanine. So, UUU turned out to be the RNA codon for phenylalanine. Other RNA codons include UAU, which codes for the amino acid tyrosine; CAC, which codes for histidine; and UGG, which codes for tryptophan.
A total of 64 three-letter codons can be formed from the four letters of the DNA bases, but there are only 20 amino acids. The overage allows for the possibility of an amino acid having more than one codon, and in fact most of them do. Three of the codons, UAA, UAG, and UGA, do not code for any amino acid. They act as signals for the release of the polypeptide from the ribosome, thus ending the process of assembling that polypeptide.
The genetic code is nearly universal. The same three-letter codons specify the same amino acids in most organisms that have been studied—from bacteria to human beings.
The cell in disease
Cells normally grow and reproduce in an orderly fashion and perform their tasks with remarkable efficiency. But sometimes, things go wrong. Instead of dividing in an orderly fashion, a cell may go wild and multiply without stopping, forming a tumor. A virus may take over the machinery of the cell for its own purposes, ultimately killing the cell. The genetic code may contain an error, leading to an inability to form a needed protein or to the formation of an abnormal protein.
Cancer
is a disease marked by a disorderly growth of cells. It occurs in human beings and other animals. Many cancer cells look like immature cells that have not yet begun to specialize into cells of a particular tissue. In some cancerous tissues, many nuclei are in the process of mitosis. The dividing cells eventually build up and form a tumor. Cells in the tumor may break away, invade other tissues, and form additional tumors that disrupt the function of the tissue. Many factors, including smoking, exposure to certain chemicals, or excessive exposure to X rays, may cause cells to become cancerous and begin the process of tumor formation. Some scientists believe that the causes of some types of cancer produce a change in the genetic code. The altered code is then duplicated and passed on to daughter cells.
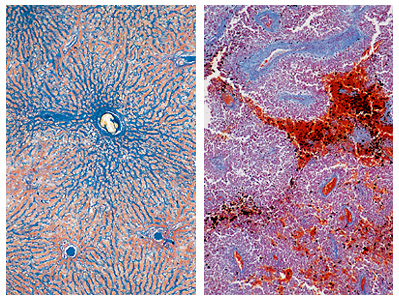
Virus diseases
occur when a virus invades a cell. Viruses are tiny parasites. They are not cells, but they share certain qualities of living things. By themselves, viruses are lifeless particles. But inside a living cell, viruses become active and capable of reproduction. Many viruses consist only of hereditary material—DNA or RNA—and various proteins. After a virus enters a cell, it may take over the cell’s machinery to produce copies of itself. When the copies of the virus are produced, the cell is sometimes destroyed. The new viruses can then invade other cells. Viruses that attack human beings cause AIDS, chickenpox, colds, flu, hepatitis, measles, mumps, poliomyelitis, and many other diseases. It is also known that certain viruses cause cancer in laboratory animals.
Metabolic diseases.
Metabolism is the sum of the chemical processes by which all living things transform food into living matter and energy. Metabolism depends on specific enzymes, which are made within cells according to the genetic code. Sometimes the code contains an error that may cause a metabolic disease. Many of these errors are inherited and are brought about by a mutation (change) in the code. Most mutations are caused by damage to the DNA through exposure to radiation or chemicals. But mutations may also happen randomly when cells are growing and dividing
Several metabolic diseases occur because of the lack of a needed enzyme. For example, galactosemia, a disease of infants, is caused by a lack of the enzyme needed to convert galactose, a milk sugar, to glucose. Phenylketonuria, another disease of infants, is caused by the lack of the enzyme needed to convert the amino acid phenylalanine to the amino acid tyrosine. Both these diseases cause intellectual disability and poor physical development.
Some metabolic diseases are caused when the instructions for making a protein are “misspelled” in the genetic code. Sickle cell anemia, a potentially fatal human disease, is one such metabolic disease. Normal red blood cells are disk-shaped. But in a sickle cell victim, some red blood cells become twisted into a hooked, or sickle, shape. These deformed cells die quickly, causing severe anemia. Red blood cells contain a protein called hemoglobin, which carries oxygen to the body’s tissues. Hemoglobin is made of several hundred amino acids. The deadly sickling occurs when, in only one part of this long chain, the genetic code calls for the amino acid valine instead of glutamic acid.
Cell research
In 1665, Robert Hooke, an English scientist, observed a thin slice of cork under his microscope. He saw that it was composed of neat holes enclosed by walls. He called these holes cells. Other scientists also studied cells and tiny living things under the microscope. But for many years, few guessed the significance of the cell.
In 1838, the German botanist Matthias Schleiden stated that the cell was the basic unit of all life. The next year, Theodor Schwann, a German physiologist, advanced the same idea. A number of other scientists had already come to believe that all organisms were made up of cells. But from that time on, biologists regarded the cell as the building block of living things.
In the mid-1800’s, Gregor Mendel, an Austrian monk, discovered laws of heredity through experiments with garden peas. Mendel’s work, translated into modern terms, suggested that there is a basic unit of heredity—the gene. Mendel’s work also suggested that the genes in a cell’s nucleus usually occurred in pairs, with each parent supplying one member of every pair. In 1865, Mendel published a paper presenting his findings. But his work went unrecognized until 1900.
During the mid- and late 1800’s, using microscopes with better lenses, scientists discovered much about cells. They learned that cells reproduce by division. They found that every cell nucleus contains a substance they called chromatin. During cell division, the chromatin condenses into a certain number of visible pairs of chromosomes, depending on the organism. Each somatic daughter cell receives the same number of chromosomes that the parent cell has. Egg and sperm cells receive only half the number of chromosomes that the somatic cells have.
Near the end of the 1800’s, a number of scientists argued that chromosomes must be the basis of heredity. However, this opinion was not yet generally accepted
The 1900’s.
Mendel’s work was rediscovered on three separate occasions in 1900—by Hugo de Vries of the Netherlands, Carl Correns of Germany, and Erich von Tschermak of Austria. Each of these botanists, working independently on the problem of heredity, came across Mendel’s findings. In 1902, Walter S. Sutton, an American scientist, pointed out that during cell division chromosomes behaved as Mendel had believed inherited traits behaved. A few years later at Columbia University, Thomas Hunt Morgan and his associates proved that genes are the units of heredity. They also proved that genes are arranged in a specific order on chromosomes.
Attention then turned to the question of how genes determine the structure and behavior of living things. Two American scientists, George W. Beadle and Edward L. Tatum, found part of the answer in the early 1940’s. They discovered that some genes control chemical reactions in cells by directing the formation of enzymes. They found that there is a specific gene for each enzyme.
Scientists became increasingly interested in the chemistry of the gene in the 1940’s. They knew that chromosomes consisted of DNA and protein. In fact, DNA had been discovered in 1868 by a Swiss biochemist, Friedrich Miescher. But scientists had dismissed DNA as unimportant, knowing how essential proteins were in life processes. The turning point came in 1944, when a team headed by American geneticist Oswald T. Avery found evidence that DNA alone determined heredity.
In 1957, Arthur Kornberg, an American biochemist, produced DNA molecules in a test tube. He did this by mixing DNA nucleotides with an enzyme and adding a chain of natural DNA as a template. The DNA nucleotides linked into a chain resembling the template DNA. Ten years later, Kornberg manufactured DNA that was biologically active—that is, able to reproduce naturally.
Many scientists have worked on figuring out the genetic code, found in the sequence of the bases of DNA. In 1962, Marshall W. Nirenberg, an American biochemist, discovered the code for one amino acid. He and others eventually determined the code for the 20 amino acids involved in protein production. Other scientists discovered how RNA copies of the DNA code are produced.
In the 1970’s, scientists discovered techniques for removing or copying genes from one organism and inserting them into another. Today, these techniques are called recombinant DNA technology. Experiments with recombinant DNA have helped scientists to learn more about the structure and function of genes and have led to advances in agriculture, medicine, and industry. See Genetic engineering.
In the 1980’s, scientists began using a powerful microscope called the scanning tunneling microscope. This tool provided scientists with detailed images of the structure of DNA within cells.
In the 1990’s, doctors began to use gene therapy as treatment for certain diseases. This treatment involves inserting a gene into the cells of a patient to correct defects in cell function. Scientists first used this method to treat patients who lacked a working immune system. For such people, exposure to common cold viruses and simple bacterial infections can be fatal. The first gene therapy treatments for this problem were successful, but later uses of this method encountered some serious problems. However, scientists are hopeful that using refined procedures, gene therapy can help to treat some disorders in patients with no other treatment options.
Stem cell research.
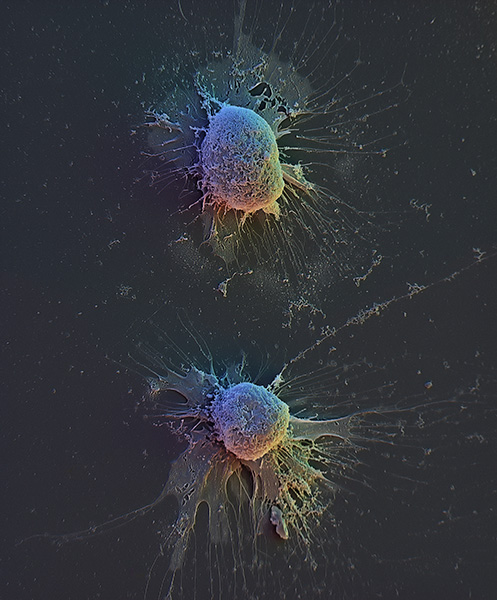
Most of the cells in the human body have taken on specific functions, losing the ability to divide. In some parts of the body, however, a small number of cells known as stem cells retain the ability to divide and replenish lost cells, even in adults. Stem cells enable the body, for example, to replace red blood cells when they die. They help replace skin cells lost in normal daily activities and to make repairs to heal a wound. But there is a limit to what these stem cells can do. In humans, for example, we know that stem cells cannot normally replace entire parts of the body like arms or legs. Other organisms, like certain lizards, can grow entire new limbs when they are lost. Scientists are studying ways to use stem cells to treat diseases caused by damaged tissues.
Synthetic cells.
In 2010, scientists announced the creation of what they called a synthetic cell. The scientists first used chemicals to construct an artificial copy of the DNA code of a bacterium called Mycoplasma mycoides. They then inserted this DNA into the cell of a different bacterium from which the DNA had been removed. The new DNA went on to replicate and caused the cell to divide, forming new cells of M. mycoides. Scientists believe this achievement is an important step toward the goal of creating true artificial cells.