Heredity is the passing on of biological characteristics from one generation to the next. The process of heredity occurs among all living things—animals, plants, and even such microscopic organisms as bacteria. Heredity explains why a human mother always has a human baby and why a mother dog has puppies—not kittens. It is also the reason offspring look like their parents.
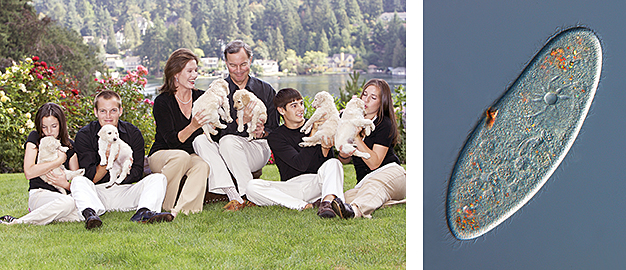
Through heredity, living things inherit characteristics, often called traits, from their parents. You resemble your parents because you inherited your hair color, nose shape, and other traits from them. All organisms consist of cells. Tiny biochemical units called genes inside each cell carry traits from one generation to the next. Genes are made of a chemical called DNA (deoxyribonucleic acid). They are found in long chains of DNA in structures known as chromosomes.
Genes are like blueprints for building a house, except that they carry the plans for building cells, tissues, organs, and bodies. They have the instructions for making the thousands of chemical building blocks in the body. These building blocks are called proteins. Some proteins are responsible for the size, shape, and structure of the parts making up your body. Other proteins, known as enzymes, make possible the thousands of chemical reactions that occur constantly in your body and in all other living things. The process by which the cell makes a protein according to the instructions carried by a gene is known as gene expression.
Genes have powerful effects, but they do not control all of life. Most characteristics result from a combination of heredity and environment. For example, you may have inherited a talent for playing the piano. But you will not be able to play unless you take lessons and practice. The talent is hereditary. The lessons and practice are environmental.
The basic laws of heredity were formulated during the mid-1800’s by an Austrian botanist and monk named Gregor Mendel. Mendel based his laws on his studies of the inheritance patterns of garden peas. Although Mendel published the results of his experiments in 1866, his work went unnoticed until 1900.
Mendel’s experiments laid the foundation for the scientific study of heredity, called genetics. Through the years, geneticists—that is, people who study heredity—have learned much about why human beings and other living things look and behave the way they do. These scientists have also begun to uncover the causes of hereditary diseases and to develop ways to treat them. Today, genetics has several specialized branches. Molecular genetics, for example, involves the study of the chemical nature and activities of genes. For more information on the various branches of genetics, see Genetics.
Chromosomes and genes
The structure of chromosomes and genes.
In human beings and many other organisms, most chromosomes are found in the part of a cell known as the nucleus. Chromosomes are tiny threadlike structures made largely of DNA and proteins. Chromosomes generally occur in pairs. The two chromosomes in a pair resemble each other in size and shape. They also contain similar hereditary information.
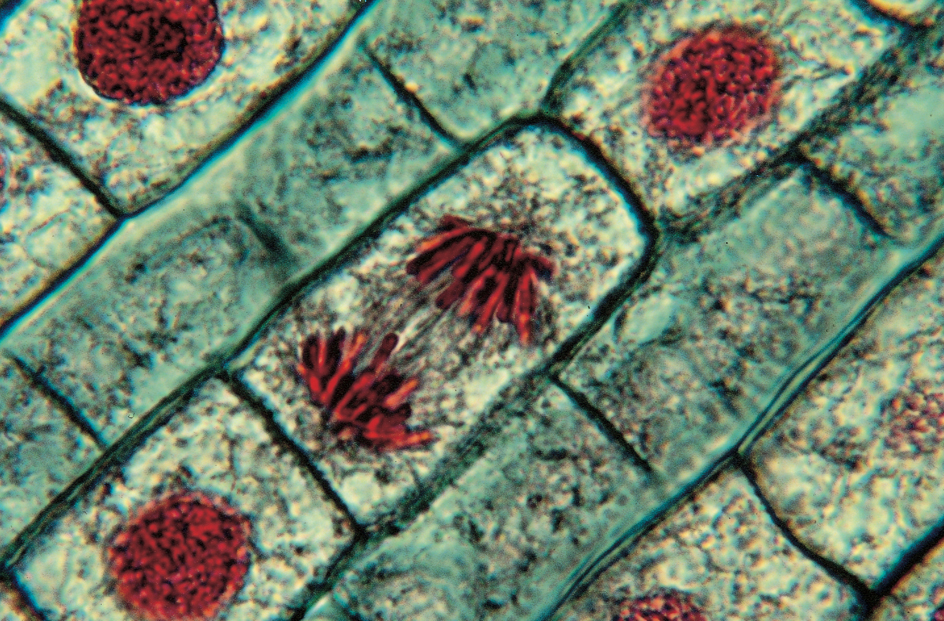
Each species of animal and plant has a characteristic number of chromosomes in its body cells. Body cells, often called somatic cells, are the cells that make up such body parts as muscles and bones. Body cells differ from sex cells. In male animals, the sex cells are sperm; in plants, pollen. In female animals and plants, the sex cells are eggs. Human beings typically have 46 chromosomes, arranged in 23 pairs, in their body cells. Dogs have 78 (39 pairs), and corn has 20 (10 pairs). The fruit fly Drosophila melanogaster, which is widely used in genetic research, has only 8 chromosomes (4 pairs).
Genes are the basic units of heredity. Each gene consists of a section of an extremely long DNA molecule found in a chromosome.
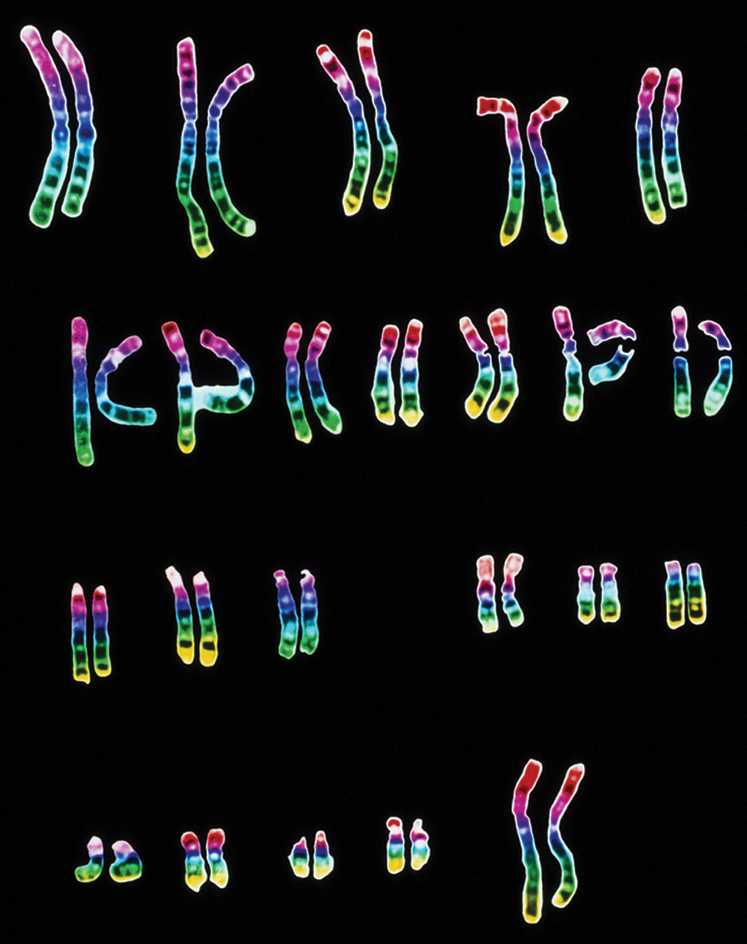
Every species of plant and animal has a certain number of genes in its chromosomes. For example, scientists estimate that human beings have 20,000 to 30,000 different genes. Roundworms may have about 18,000 different genes, and fruit flies may have about 13,000. A set of all the genes a species has in its chromosomes is called its genome.
Sex cells and reproduction.
There are two general types of reproduction, asexual and sexual. Asexual reproduction involves only one parent. The offspring have chromosomes identical to those of the parent. When a worm called a planarian reproduces asexually, for example, its body divides into two sections. One has the head, and the other has the tail. Each section grows the parts that are missing and becomes a new individual that is genetically identical to its parent.
Sexual reproduction generally involves two parents, each of which contributes half the chromosomes to the offspring. Sexual reproduction starts with the production of specialized sex cells that are called gametes. Gametes—that is, sperm, pollen grains, and eggs—are produced in a process of cell division called meiosis. Meiosis results in the sex cells’ having half the number of chromosomes found in the body cells. In human beings, therefore, meiosis produces sperm and egg cells that have 23 chromosomes each. In dogs, the number of chromosomes in each sex cell is 39.
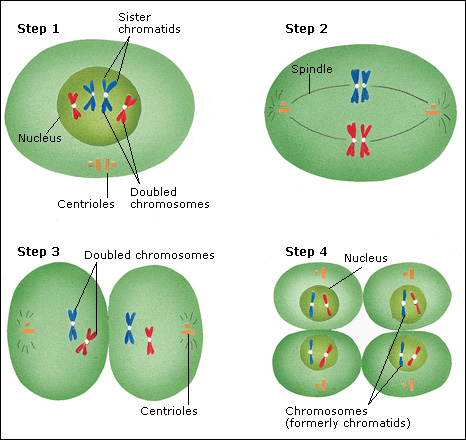
The uniting of an egg cell and a sperm cell, called fertilization, restores the full number of chromosomes. In human beings, the resulting cell, known as a fertilized egg, has 46 chromosomes, 23 pairs. One chromosome of each pair comes from the mother’s egg, and the other from the father’s sperm. After the egg has been fertilized, it begins to divide and produce exact copies of itself by a type of cell division called mitosis. Each of the new cells, known as daughter cells, has the same number of chromosomes as the original cell. For more information on meiosis and mitosis, see Cell (Cell division).
Chromosomes and sexual determination.
In human beings, chromosomes known as X and Y chromosomes determine an individual’s sex. Eggs always have an X chromosome, but sperm may have either an X or a Y chromosome. When a Y-carrying sperm fertilizes an egg, the baby will be a boy (XY). If the sperm has an X chromosome, then the baby will be a girl (XX). Males produce sperm with an X chromosome in numbers equal to sperm with a Y chromosome. As a result, about half of all babies are boys, and half are girls.
Patterns of heredity
Dominant and recessive genes.
Most genes occur in pairs. Each pair of genes is contained in a pair of matching chromosomes, with one copy of a gene in each chromosome. Some hereditary traits are determined by a single pair of genes. For example, a chemical called phenylthiocarbamide (PTC) tastes extremely bitter to some people. Other people cannot taste PTC at all. The difference between tasters and nontasters is due to a single pair of genes. But many other traits, called polygenic traits, are influenced by a number of pairs of genes. Tens or hundreds of pairs of genes are involved in the inheritance of such traits as height, weight, and intelligence.
The two genes in a pair may differ in the effects they produce. Different forms of the same gene are called alleles. Some alleles are dominant, and others are recessive. A dominant allele masks the effects of its recessive partner. In other words, the dominant allele is expressed, and the recessive allele is not. A trait that results from a recessive allele is evident only in an individual that has two recessive alleles for that trait. For example, Mendel showed that in pea plants the allele that produces violet flowers (symbolized by V) is dominant over the one that causes white flowers (v). Pea plants that have two dominant alleles for violet flowers (VV) or one allele for violet flowers and one for white flowers (Vv) will have violet flowers. Only those with two recessive alleles (vv) will have white flowers.
Sex-linked genes.
Genes that lie near each other on the same chromosome are called linked genes because they tend to be inherited together. Genes on the sex chromosomes are called sex-linked genes. Human beings have more than 250 genes on the X chromosome—called X-linked genes—that can cause hereditary disorders. These disorders include hemophilia and a type of muscular dystrophy. Most of these disorders are recessive. They typically occur only in males because males have only one X chromosome. Females have two X chromosomes. Generally, at least one of the two X chromosomes has the dominant normal allele. This allele determines the trait, and the harmful gene on the other X chromosome does not.
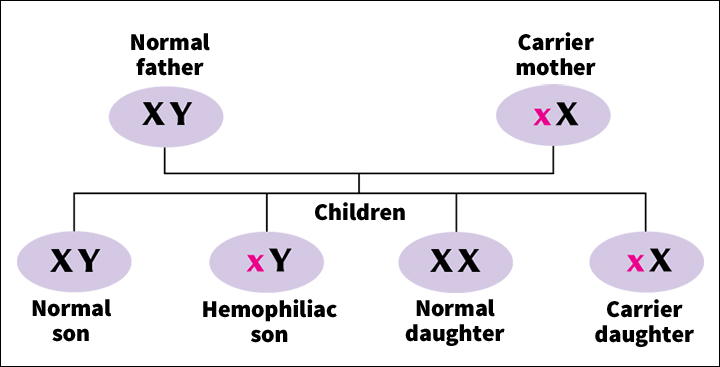
Sources of genetic variation
Individual members of a species differ widely from one another in their genetic makeup and therefore in their traits. You may look like your parents, but you are not an exact duplicate of either of them. You inherited half your genes from your father and half from your mother. Nor do you look exactly like your classmates, even though you and your classmates are all human beings. Scientists refer to the differences among members of a species as genetic variation. This section discusses the main sources of diversity among individual members of a species—mutation, independent assortment, and genetic recombination. The next section, Heredity and natural selection, describes how entire species and certain groups within species change over time.
Mutation
is a permanent change in the amount, structure, or sequence of the DNA in an organism’s cells. It can result in alterations in gene expression that can change traits.
Kinds of mutations.
Mutations can occur in sex cells or in body cells. A germinal mutation affects the DNA in the gametes and is therefore passed on from an organism to its offspring. A somatic mutation occurs in body cells. In human beings and other animals, somatic mutations do not affect the gametes. Consequently, the changes are not inherited by succeeding generations. However, the changes are passed along to the daughter cells of the original mutant cell.
Many mutations affect entire chromosomes. In some cases, an organism has too many or too few chromosomes. For example, people with a condition called Down syndrome have an extra copy of chromosome 21, one of the 23 pairs of chromosomes. Sometimes the structure of a chromosome is abnormal. For example, a mutation called translocation occurs when part of one chromosome breaks off and attaches to another.
Some mutations affect only one or a few of the chemical units making up the DNA molecule. There are several kinds of these point mutations or gene mutations. For example, a missense mutation changes a single pair of chemical units, thereby altering the instructions carried by that segment of DNA.
Causes of mutations.
Some mutations result from mistakes that occur when copies of DNA are made during cell division. Others are caused by agents called mutagens. Mutagens include certain chemicals and various forms of radiation.
Independent assortment
refers to the way in which chromosomes and their genes are distributed when a sex cell divides to form eggs or sperm. An immature sex cell contains two of each chromosome—one from the individual’s father and one from the mother. During meiosis, each pair of chromosomes separates, and each egg or sperm receives one chromosome from each pair. Because the chromosomes separate in a random manner, each egg or sperm receives some chromosomes from the individual’s mother and some from the father. This reshuffling of chromosomes and genes can result in new combinations of traits in offspring.
Genetic recombination
is the exchange of genes between two partner chromosomes. Genes on separate chromosomes are inherited in a random and independent manner. But genes located close to each other on the same chromosome are generally inherited together. In other words, genes that are closely linked on a parent’s chromosome largely remain linked in offspring.
Sometimes, however, linked genes are not inherited together. This situation arises because of crossing over. Just before immature sex cells divide to form sperm or eggs, each chromosome of a pair lines up side by side with its partner chromosome. During a crossing-over event, groups of genes from one chromosome change places with groups of genes from its partner chromosome. As a result, different sperm or eggs may carry different combinations of linked genes.
Heredity and natural selection
Mutation is one of the chief sources of new genetic material for a species or a population—a group of individuals of the same species living in the same area. Independent assortment and genetic recombination provide new combinations of mutations. As these sources of genetic variation work together with a process called natural selection over time, the genetic makeup of a species or population changes.
Natural selection.
Many mutations that alter traits are harmful, but some mutations and combinations of mutations make certain individuals better able to survive, mate, and reproduce in a given environment. These individuals will likely have more offspring that survive than individuals without the particular genetic variation. The young of the better-suited parents inherit the genes for the favorable characteristics that enabled their parents to improve their chances of surviving, mating, and reproducing. They then pass the genes along to their own offspring. As this process is repeated through many generations, more and more members of the species or population come to have the favorable genes. In this way, the process of natural selection changes the genetic makeup and therefore the traits of organisms through time.
Generally, evolution by natural selection takes place slowly and is not noticeable in a human lifetime. However, a species may change quickly in response to a major alteration in the environment, such as pollution or the introduction of a pesticide.
An example of a rapid genetic change occurred in Britain during the 1800’s with a species of moth called the peppered moth. Peppered moths rest on light-colored lichens that grow on tree trunks. During the early 1800’s, nearly all peppered moths were light-colored, and only a few moths carried a mutation that made them black. In the mid-1800’s, however, factories burned so much coal that soot settled over the countryside, killing the lichens and blackening the tree trunks. Light-colored moths on dark-colored trees were easily seen and eaten by birds. But the birds could not see the black moths well. As a result, more of the black moths survived and produced offspring. Within 50 years, most moths in heavily polluted areas were black. After air pollution laws were passed in the mid-1900’s, the tree trunks became lighter as lichens again grew on them, and the number of light-colored moths increased.
Gene pools and allele frequency.
The genes of all the individuals of a population are called a gene pool. The degree to which a particular allele is present in a population is called the allele frequency. The level of diversity in a gene pool is important because genetic variation is necessary for a population to adapt to changes in the environment. For example, an insect population that has a genetic variant for resisting a new pesticide in its environment will be more likely to survive. There will be strong selection for that variant, and its frequency in the gene pool will increase. On the other hand, an insect population that does not have such a variant will die off.
Several factors besides natural selection may influence the frequency of particular alleles in a gene pool. These factors include gene flow and genetic drift.
Gene flow
is the transfer of genes from one population to another. When separate populations come into contact and interbreed, new genes or combinations of genes are introduced into each group. Consequently, the gene pool of each group comes to include genes from pools of the other populations. In this manner, the allele frequencies of populations may change over time.
Genetic drift
refers to chance increases or decreases in allele frequencies of a population from generation to generation. The genes of each generation represent only a sample of the previous generation’s gene pool. As a result, the allele frequencies of each generation of individuals tend to vary randomly within the limitations of the preceding generation’s gene pool. Such changes will probably have little effect on each generation in large populations. But they can lead to major genetic changes in small ones in a short period.
For a detailed discussion of the factors involved in genetic variation among human populations, see Races, Human (How human populations develop and change).
Hereditary disorders
Many diseases and disorders are caused by such agents as viruses and bacteria. In other cases, however, the cause of the problem is hereditary—that is, the organism has inherited one or more abnormal genes from its parents. Because genes carry chemical instructions for the formation of proteins, defective genes can affect the production and function of proteins. For example, hemophiliacs are born with a defective gene, and thus they are unable to produce a protein crucial for blood clotting. As a result, they suffer prolonged bleeding when they are injured because their blood clots slowly.
Genes are arranged in an exact order along the length of the chromosomes. Researchers use a process known as gene mapping to locate and identify genes on a chromosome. This process has helped scientists determine which genes are responsible for some hereditary conditions. For example, Huntington’s disease, a severe disorder of the nervous system, is caused by an abnormal gene on chromosome 4.
Many diseases, such as diabetes and rheumatoid arthritis, are common in certain families. These diseases are therefore believed to have a hereditary basis. People inherit a tendency to develop these conditions, but not the diseases themselves. Environmental factors may play a role in whether the conditions actually develop. For example, people with a genetic tendency toward diabetes may increase their chance of getting the disease by overeating and not getting enough exercise.
Scientists have developed methods of treating some hereditary disorders. For example, hemophilia can be treated by injections of the clotting factor that is missing from the blood of people who have the condition.
A technique known as gene therapy may offer a way of treating hereditary disorders and certain other diseases. Such therapy involves identifying the gene that causes a genetic disease and then supplying patients with a normal copy of that gene. The normal genes come from another individual or organism and are usually inserted into a patient’s cells outside the body. The altered cells are then returned into the patient’s body. See Gene therapy.
Couples who are planning to have children can be tested to see if they carry certain potentially harmful genes. These tests are usually done as part of a process called genetic counseling. This process enables couples to understand their chances of bearing children with hereditary disorders. It also helps them learn ways of dealing with their situation. See Genetic counseling.
Heredity and environment
A gene gives only the potential for the development of a trait. How this potential is achieved depends partly on the interaction of the gene with other genes. But it also depends partly on the environment. For example, a person may have a genetic tendency toward being overweight. But the person’s actual weight will depend on such environmental factors as how much and what kinds of food the person eats.
The underlying genetic makeup of a trait is called the genotype. The actual appearance of the trait is known as the phenotype. Sometimes the word phenotype is used to refer to the overall appearance of an individual; genotype, to refer to the person’s overall genetic makeup.
Scientists have long debated the relationship between heredity and environment in determining a person’s physical appearance and behavior. This debate is often referred to as the question of nature versus nurture—that is, heredity versus environment.
To try to understand the influence of genetics and the environment on phenotype, researchers have often studied identical twins, who have the same genotype. Such studies have shown that identical twins raised apart and thus in different environments tend to vary more in their characteristics than identical twins raised together. As a result, scientists have concluded that both heredity and environment play important roles in what an individual’s ultimate phenotype will be.
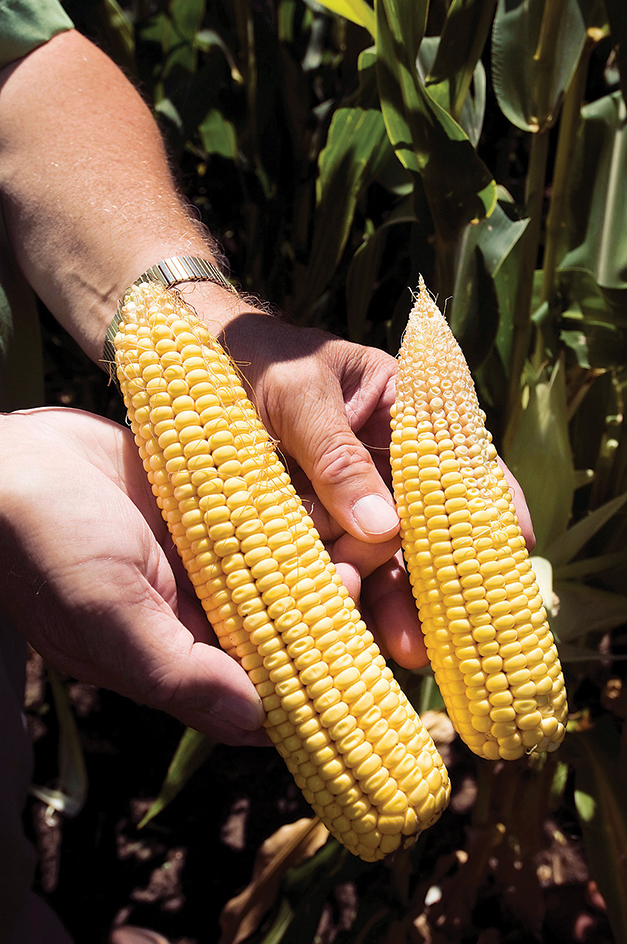
Intelligence and other mental traits also depend on heredity and environment. Every person is born with a certain mental capacity that influences how intelligent he or she will be as an adult. The development of this capacity is influenced by the person’s environment. For example, severely undernourished infants may fail to develop their natural abilities.
The flow of genetic information
Genes and proteins.
Genes carry the instructions for producing proteins. Proteins are large, complex molecules made up of smaller units called amino acids. Twenty kinds of amino acids are commonly found in proteins. Various combinations of these amino acids are linked to form long chains known as polypeptides. Polypeptide chains fold into complex three-dimensional shapes.
Proteins consist of one or more polypeptide chains. These chains vary greatly in length, from only a few amino acids to thousands. They also differ according to the order in which the amino acids occur. The length of the polypeptide chains and the arrangement of their amino acids determine the protein’s shape and function. In most cases, one gene is responsible for making one polypeptide. The gene determines the order and number of amino acids in the polypeptide and thus the shape and function of the protein.
Proteins are found in every cell and are essential to plant and animal life. The proteins called enzymes speed up the chemical processes of life. Without enzymes, most of the reactions that occur in living things would happen too slowly to make life possible. These reactions include breaking down food during digestion and burning carbohydrates and fat for energy.
Many other proteins serve as building blocks for cells. Cells have different sizes and shapes—and different kinds, numbers, and arrangements of proteins—depending on where they are in an organism. Hair, nails, and part of the skin are made of a tough protein called keratin. The red color in blood comes from a red protein called hemoglobin that carries oxygen from the lungs to all parts of the body. Muscles consist largely of two proteins called myosin and actin.
The structure of DNA.
The genes of all living things except some viruses are composed of DNA, which is often referred to as the hereditary material. DNA is a thin, chainlike molecule made up of smaller chemical units called nucleotides. A nucleotide in DNA is composed of a sugar known as deoxyribose, an oxygen-phosphorus chemical group called a phosphate, and a nitrogen-containing compound known as a base. The sugar and phosphate are the same in all DNA nucleotides, but the bases vary. There are four DNA bases: adenine (A), guanine (G), thymine (T), and cytosine (C).
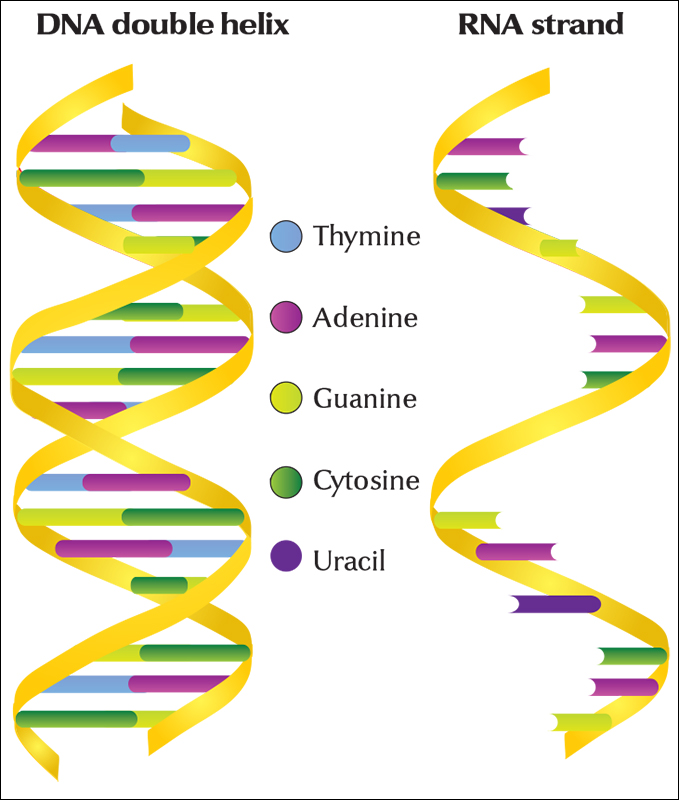
DNA consists of two chains that coil around each other in a shape called a double helix. The double helix resembles a twisted ladder. The sides of the ladder consist of the linked sugars and phosphates of the nucleotides. Each rung is made up of two paired bases.
Base pairs.
The bases of a DNA molecule are paired in a specific way to form combinations known as base pairs. There are A-T and G-C base pairs. Wherever there is adenine in one strand, there is thymine in the opposite strand. Wherever there is guanine in one strand, there is cytosine in the other. Because of this specific pairing of the bases, scientists say the two DNA strands are complementary. The sequence of bases in one strand determines the sequence of bases in the other.
DNA molecules in human beings typically have more than 100 million base pairs and measure more than 1 inch (2.5 centimeters) long when uncoiled. However, DNA is so thin it can be seen only with the aid of special microscopes called electron microscopes. A typical DNA double helix has a thickness of only 20 angstroms. One angstrom equals 1/10 nanometer, or 1/10,000,000 millimeter (1/254,000,000 inch).
Replication.
Most of the cells in your body divide from time to time. When you get a cut, for example, the skin cells around the wound begin to divide to make new skin to repair the damage. Each of the new daughter cells produced by cell division has the same DNA as the old cells. The process by which exact copies of DNA are made during cell division is called replication. The accurate replication of DNA is one of the essential characteristics of the hereditary material. Without it, daughter cells would be genetically different from each other and from the parent cell. Your genetic makeup, and therefore your physical characteristics, would be constantly changing as your cells divided.
The complementary nature of the two DNA strands enables a cell to make exact copies of its DNA. Before the cell divides, sections of the two original paired strands of a DNA double helix separate lengthwise, breaking the base pairs. This process is similar to what would happen if a ladder split down the middle, separating its sides. Each of the DNA strands, which resembles a half ladder, then picks up free nucleotides from the cell nucleus. The bases in the free nucleotides, with their attached sugars and phosphates, pair with the matching bases on the original DNA strands. A’s pair with T’s, T’s with A’s, G’s with C’s, and C’s with G’s. In this way, two double-stranded DNA ladders are produced that have exactly the same base sequence as the parent DNA. When the cell divides, each of the new daughter cells receives identical DNA molecules.
How proteins are made.
The genetic code is a code formed by the sequence of the bases in DNA. This code tells the cell how to put amino acids together in the right order to make a specific polypeptide.
A group of three bases in a certain order forms a unit of the genetic code called a codon. Most codons specify a certain amino acid. The order of codons in DNA determines the sequence of amino acids in a protein—and the protein product that is made.
A total of 64 three-letter codons, often called triplets, can be formed from the four letters of the DNA bases. Because there are only 20, not 64, amino acids, there is more than one codon for most amino acids. In addition, with only minor exceptions, the same three-letter codons specify the same amino acids in all organisms that have been studied. Therefore, the genetic code is nearly universal.
Gene expression, the process by which the cell makes a protein according to the instructions carried by a gene, takes place in two steps. These steps are known as (1) transcription and (2) translation.
Transcription
involves a close chemical cousin of DNA called RNA (ribonucleic acid). Like DNA, RNA is a nucleic acid and is composed of nucleotides. But the sugar in RNA is ribose instead of deoxyribose, and RNA contains the base uracil (U) instead of thymine. Like thymine, however, uracil base-pairs with adenine.
During transcription, a cell makes an RNA copy of one of the DNA strands of a gene. Part of the twisted DNA ladder unwinds and splits. One of the half ladders then serves as a template (mold) for lining up the RNA bases. The bases of the free nucleotides pair with the exposed DNA bases. For example, the RNA bases CUACAG pair with the DNA bases GATGTC. An RNA strand called messenger RNA (mRNA) is formed, and it is a complementary copy of the DNA blueprint.
As transcription proceeds, the mRNA copy of the gene peels off the DNA template. It then carries the instructions for making the gene’s protein product to the cell’s protein factories, which are structures known as ribosomes. The ribosomes are outside the nucleus in a part of the cell called the cytoplasm. The mRNA acts as a guide to line up the amino acids in the order called for by the gene, forming the correct protein chain.
Translation.
In the translation step of gene expression, the ribosomes “read” the code in the mRNA and link amino acids in the order the mRNA codons dictate. Another type of RNA, called transfer RNA (tRNA), plays a key role in translation. Transfer RNA brings amino acids to the ribosomes from other parts of the cytoplasm.
A molecule of transfer RNA has two sites with important roles in translation. One site contains an anticodon, which consists of three nucleotides. The bases in the nucleotides of each tRNA anticodon are complementary to those in an mRNA codon and can therefore base-pair with them. The other site is at one end of the tRNA molecule. The tRNA binds to a specific amino acid at this site. For example, tRNA with the anticodon CGU binds to the mRNA codon GCA, which codes for the amino acid alanine. The end of the tRNA binds to alanine. Thus, this tRNA can deliver alanine to the ribosome.
The mRNA moves through a ribosome one codon at a time. After a codon arrives at the ribosome’s decoding center, a tRNA molecule brings in the appropriate amino acid. In this way, the polypeptide grows, one amino acid at a time, until the ribosome reaches the end of the message in the mRNA. The last codon of the message signals that the chain is complete and tells the ribosome to stop production.
After the ribosome reads the stop codon, the finished polypeptide chain is released. The ribosome can then produce another polypeptide. In most proteins with more than one polypeptide chain, the chains are made separately. They then combine to make the protein.
History of the study of heredity
Early ideas about heredity.
The Greek philosopher Aristotle (384-322 B.C.) proposed one of the oldest known theories of inheritance. He taught that traits are inherited through the blood. This theory was wrong, but it was generally accepted for more than 1,000 years.
A correct theory of inheritance could not be formulated until after sex cells had been discovered and their functions determined. The discovery of eggs and sperm occurred during the late 1600’s. At that time, many biologists thought that either the egg or the sperm contained a tiny but fully formed embryo that merely increased in size inside the mother. During the late 1700’s, Caspar Friedrich Wolff, a German scientist, proved embryos are not preformed. He showed that an embryo grows from a fertilized egg, and he argued that the sperm and the egg contribute equally to an embryo.
During the early 1800’s, a French biologist and nobleman, the Chevalier de Lamarck, suggested that traits acquired during the lifetime of an organism could be passed on to its offspring. Later genetic discoveries showed that transmission of such acquired characteristics from one generation to the next does not occur.
However, Lamarck’s theory was basically accepted by the British biologist Charles Darwin, who proposed the theory of natural selection in his book The Origin of Species (1859). Darwin believed each part of the body produced tiny particles that moved through the bloodstream into the eggs or sperm. These particles supposedly influenced hereditary traits. The British scientist Francis Galton, a cousin of Darwin’s, disproved this idea. Galton transfused blood from black rabbits into white rabbits to see if the white ones would have black babies. But the white rabbits still produced white offspring.
Mendel’s laws.
In the mid-1800’s, Gregor Mendel, an Austrian botanist and monk, conducted a series of experiments on inheritance. In his monastery garden, he studied traits in pea plants. These studies led Mendel to formulate the first correct theory of heredity. His theory had two principles called Mendel’s laws of heredity.
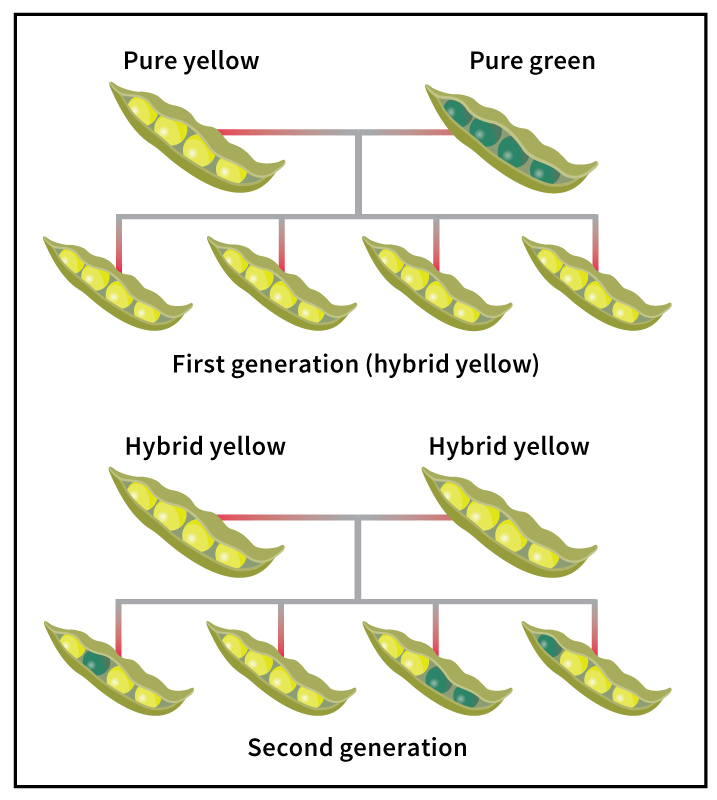
Mendel’s first law, the Law of Segregation, has three parts. (1) Hereditary characteristics are determined by separate units (now called genes). (2) These units occur in pairs. (3) The genes in a pair segregate (separate) during the division of sex cells, and each sperm or egg receives only one member of the pair.
Mendel’s second law is called the Law of Independent Assortment. It states that each pair of genes behaves independently of all other pairs in the production of sex cells. Therefore, each gene pair is inherited independently of all other genes. Geneticists now know that independent assortment applies only to genes that are on different chromosomes or far apart on the same chromosome. Genes that are linked, or near each other on the same chromosome, tend to be inherited together.
The birth of genetics.
Mendel published a report of his work in 1866. But it went unnoticed until 1900. Then, three European botanists studying heredity each rediscovered Mendel’s work. These three scientists were Hugo de Vries of the Netherlands, Carl Correns of Germany, and Erich von Tschermak of Austria. They conducted plant-breeding experiments and independently got results similar to those Mendel had obtained.
Many important genetic discoveries quickly followed the rediscovery of Mendel’s principles. In the early 1900’s, a group of scientists led by Thomas Hunt Morgan at Columbia University in New York City discovered many important aspects of heredity. These researchers included Calvin B. Bridges, Hermann J. Muller, and Alfred H. Sturtevant. Morgan and his team studied the inheritance of such traits as eye color and wing shape in fruit flies. They showed that genes were on chromosomes, made the first genetic map, demonstrated the inheritance of genes on sex chromosomes, and discovered crossing over. In 1931, the American geneticist Barbara McClintock demonstrated that crossing over involves the physical exchange of chromosomal material.
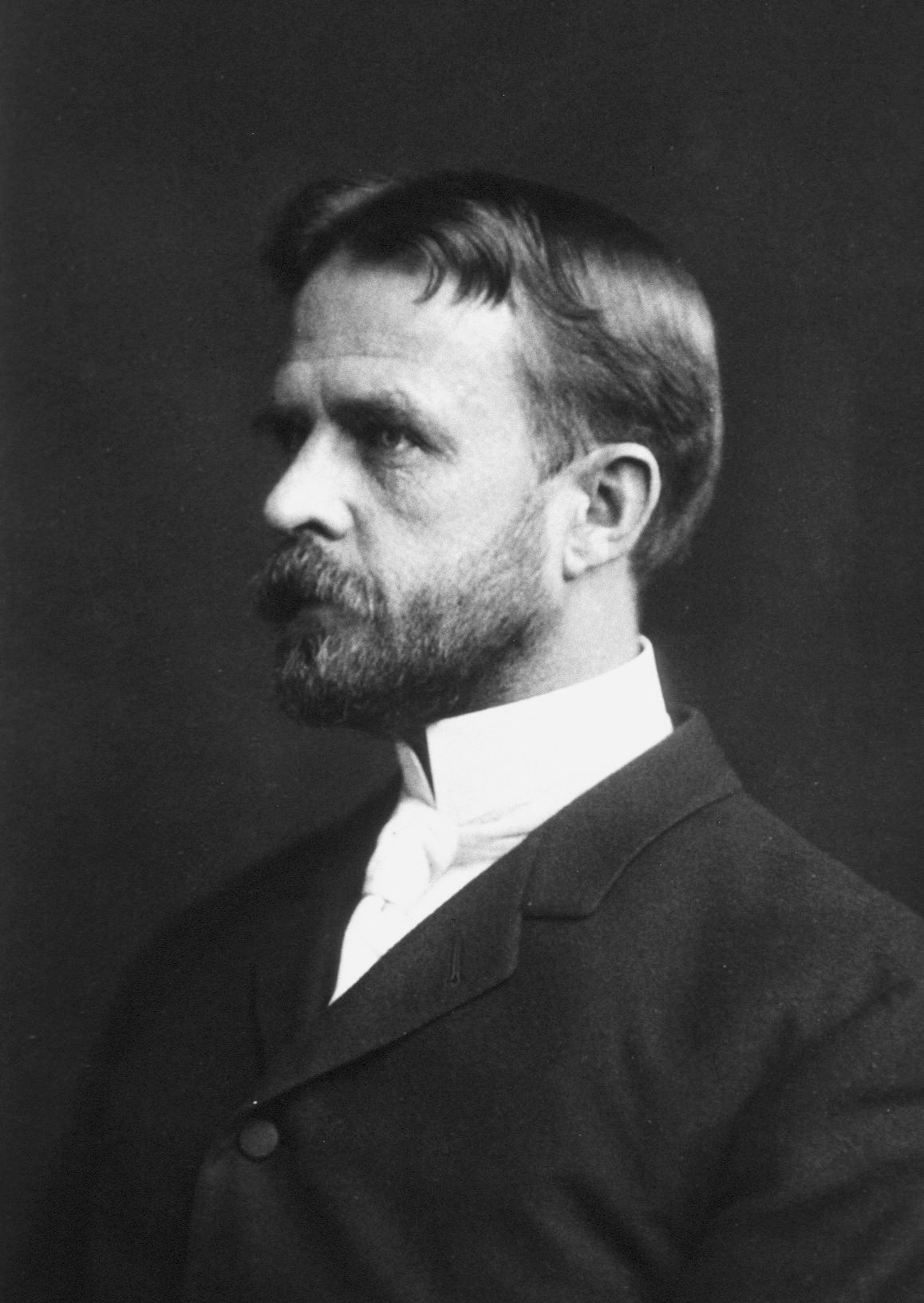
The chemistry of genes
was the focus of much genetic research after 1940. By the early 1950’s, scientists had proved genes control chemical reactions in cells by directing the production of enzymes and other proteins. They had also found that DNA is the hereditary material.
Two scientists, James D. Watson of the United States and Francis H. C. Crick of the United Kingdom, proposed a model of the ladderlike chemical structure of DNA—the double helix—in 1953. Their model was a breakthrough because it suggested for the first time how DNA can be replicated faithfully. Watson and Crick suggested that DNA replicates by splitting down the middle and building two ladders by matching complementary halves. They also proposed that mutations result from a change in the sequence of bases along the ladder. In 1958, the American geneticists Matthew S. Meselson and Franklin Stahl demonstrated experimentally that DNA replicates just as Watson and Crick had proposed.
In 1961, scientists working at the California Institute of Technology reported their discovery of messenger RNA. That same year, the American biochemist Marshall W. Nirenberg and his colleagues at the National Institutes of Health (NIH) identified the first word of the genetic code—UUU. By 1967, the entire code had been broken.
The era of genetic engineering.
Since the early 1970’s, much of the study of heredity has involved the use of techniques called genetic engineering or recombinant DNA technology. Recombinant DNA technology enables us to put together genes from different organisms to make molecules called recombinant DNA.
Scientists produce recombinant DNA in several ways. In one method, they use enzymes called reverse transcriptases to make DNA copies of messenger RNA. They then construct recombinant DNA molecules by attaching these copy DNA (cDNA) to special DNA molecules called vectors. Vectors have the ability to enter cells and replicate. Next, scientists transplant the recombinant DNA into cells. When the cells reproduce, researchers can obtain many identical cells containing this DNA. A group of genetically identical cells is known as a clone, and recombinant DNA is often called cloned DNA.
In another method, scientists use enzymes called restriction enzymes to cut the chromosomal DNA from a plant or animal into pieces. They can then produce and clone recombinant DNA with these pieces by using the same basic technique they used with the cDNA.
Experiments with recombinant DNA have revealed much about gene structure and function. Recombinant DNA technology also has major medical applications. In 1982, genetically engineered insulin for diabetics became the first recombinant DNA drug approved by the United States Food and Drug Administration (FDA) for use on people. Other genetically engineered drugs followed. They include human growth hormone (hGH), which is used to treat children whose growth is seriously below average; tissue plasminogen activator, used to treat heart attacks by breaking up blood clots; and interferon, used to halt the spread of viruses from cell to cell.
Another important medical application of recombinant DNA technology is gene therapy. In 1990 and early 1991, researchers at the NIH used gene therapy on patients for the first time. They treated two young girls who had faulty genes for the enzyme adenosine deaminase (ADA). The genes produced a deficiency of the enzyme, which caused the girls to have severely weakened immune systems, a disorder known as severe combined immunodeficiency disease (SCID). The researchers used a modified virus to deliver a normal copy of the ADA gene to the girls. A few months after gene therapy, both girls had properly working immune systems. However, the therapy did not cure their condition, and the girls needed to receive periodic additional treatment. In 2000, French researchers reported the use of another cloned gene to treat a related form of SCID in two infants. After 10 months, both patients had experienced full correction of their disease.
By the mid-1990’s, teams of geneticists had identified and cloned the genes that control several other serious genetic disorders. They include amyotrophic lateral sclerosis (ALS), Duchenne muscular dystrophy, cystic fibrosis, and Huntington’s disease.
Recombinant DNA technology has agricultural applications as well. Researchers are experimenting with using genetic-engineering techniques to improve certain features of crops, including flavor, shelf life, and the ability to resist diseases and pests.
In 1996, Scottish scientist Ian Wilmut and his co-workers cloned a sheep. They destroyed the nuclei of sheep egg cells and replaced them with nuclei from sheep udder cells. The egg cells were then placed into the uterus of a female sheep. One egg developed into a lamb named Dolly. Since Dolly had the same DNA as the sheep that donated the udder cells, the two sheep were genetically identical. Using similar techniques, other geneticists have since cloned such mammals as cats, cows, mice, and pigs. The cloning of mammals has raised the possibility of cloning human beings.
The Human Genome Project.
Geneticists from around the world launched the Human Genome Project in 1990. In June 2000, the project and Celera Genomics Corporation, a private company, announced that together they had determined essentially the entire sequence of the DNA bases in the human genome. Scientists have used these findings to determine that the human genome contains about 20,000 to 30,000 genes, far fewer than previously believed. Scientists also have found that human beings share many genes with such primitive organisms as bacteria.
In addition, geneticists have obtained entire sequences of genomes for several smaller organisms. The sequence of the baker’s yeast genome, including 6,000 genes, was reported in 1996. In 1998, geneticists determined the sequence of a species of roundworm. In 2000, they unraveled the sequence of a small mustard plant. The genomes of many bacteria, including E. coli, have also been determined. Genome research has exciting applications, such as the search for genes that cause diseases. Finding such genes could enable scientists to better diagnose and cure disease.